-
The peanut, also known as Arachis hypogaea L, is a legume crop grown primarily for its edible seeds. It is native to South America but is now cultivated worldwide[1]. Known for its rich nutrient content, including proteins, fats, and various vitamins, peanuts are widely used in culinary applications, ranging from peanut butter to various snacks. They also play a role in crop rotation due to their ability to fix nitrogen in the soil[2]. Peanuts are also considered a significant oil crop due to their high oil content, which typically ranges from 36% to 56%. This makes them one of the most valuable oil seed crops globally[3]. The oil extracted from peanuts is highly regarded for its nutritional quality and stability, making it a preferred choice for cooking and food processing. Rich in monounsaturated and polyunsaturated fats but low in saturated fats, peanut oil contributes to a heart-healthy diet[4]. Additionally, the oil extraction process generates by-products like peanut meal, which is used as a high-protein animal feed, further enhancing the crop's economic value[5].
Dormancy and germination are crucial for sustaining the life cycle of the next generation[6]. Advances in science and technology have enhanced our understanding of these complex physiological processes in crops, which are influenced by genetic and environmental factors[7]. In practical agriculture, some peanut varieties have a very short dormancy period. If not harvested in time, they may germinate before being collected[8]. Conversely, other peanut varieties, such as Virginia-type groundnuts have a longer dormancy of 4 or more months[8,9].
Wild peanuts possess robust dormancy mechanisms that prevent immediate germination upon planting. To enhance germination rates, several approaches have been adopted. Employing alternating temperatures or a specific temperature regime can effectively break dormancy by simulating natural environmental conditions to which the seeds are responsive[10]. Among the methods, using chemicals like ethylene to promote germination is the most economical and convenient. Ethylene is particularly effective in overcoming physiological dormancy by activating growth hormones. Compared to breaking dormancy through temperature changes, ethylene is more effective[11], and it can also reverse the inhibition of seed germination caused by abscisic acid (ABA)[12].
Ethylene is an essential plant hormone that plays a critical role in many aspects of plant growth and development, including seed germination[13]. The application of ethylene or ethylene-releasing compounds to seeds can effectively hasten germination. This action enhances the success rate of seed sprouting in both agricultural and ecological contexts[14]. Ethylene stimulates the production of enzymes that degrade components of the seed coat, softening it and allowing the radicle (the embryonic root) to break through more easily[15]. Once the dormancy is broken, ethylene also plays a role in the growth processes of the emerging seedling. It promotes cell division and expansion, which are vital for the growth of the seedling[16]. The interaction between ethylene, ABA, and gibberellins (GA) is particularly important for seed germination, as these hormones regulate key processes that determine whether seeds remain dormant or germinate. Gibberellins promote germination by stimulating growth and weakening the seed coat, while ABA typically inhibits these processes. Ethylene serves as a modulator, shifting the hormonal balance toward germination by reducing ABA levels and enhancing GA biosynthesis or activity[17,18]. This dynamic interplay ensures that seeds germinate under favorable conditions, optimizing their chances of survival and growth.
Although ethylene has been used to break dormancy and promote successful germination in peanuts, research on its molecular mechanisms is still limited. The details of how ethylene regulates the synthesis or breakdown of abscisic acid (ABA) and gibberellins (GA), as well as how it facilitates the breakdown of fatty acids to provide energy for germination, remain unclear. Therefore, this study aims to further dissect the molecular mechanisms behind ethylene's ability to break dormancy in peanut seeds by comparing differences in ABA and GA levels, related gene expression, and fatty acid content between seeds treated with and without ethylene during the germination process.
-
Peanut seeds from the 'Huaguan' variety were used for germination experiments. These seeds were freshly harvested, fully matured, and visually healthy. They were washed with clean water to remove surface contaminants and then evenly spread on sterile gauze placed in seedling trays. Ethylene solutions of various concentrations were prepared by dissolving ethephon (CE5121, Coolaber, Beijing, China) in sterile water. The concentrations used were 0.1, 0.2, 0.4, 0.5, 1, 2, and 5 mM. Lower concentrations were obtained by serial dilution of higher-concentration ethephon solutions with sterile water. This method ensured accuracy and consistency in preparing solutions of varying ethylene levels for the experiments. The seeds were immersed in the ethylene solutions, ensuring that one-quarter to one-third of each seed was submerged. Control groups were immersed in sterile water to the same depth. To maintain humidity, the seedling trays were covered with lids. The temperature was maintained at 25 °C, and the seeds were kept in the dark. Each treatment included 100 seeds and was replicated three times, totaling 300 seeds per treatment. Samples for measuring plant hormone levels, fatty acid content, and gene expression were collected at 0 h (after 12 h of soaking in ethylene or sterile water), 4, 8, and 12 h. At each time point, 10 seeds were randomly selected from each replicate and mixed. Samples were labeled as C0, C4, C8, and C12 for the control group, and E0, E4, E8, and E12 for the ethylene-treated group. Since C0 and E0 had not yet germinated, they were combined for hormone and fatty acid measurements, representing the initial 0-h content levels.
Seed germination analysis
-
The germination rates of peanut seeds were recorded at various time points. The germination rate was calculated using the formula: Seed germination rates = Number of germinated seeds/Total number of seeds tested × 100%. A germination index was employed to comprehensively evaluate both the germination rate and speed. The germination index was calculated using the formula: Germination index = ∑(Gt/Dt), where Gt is the number of seeds germinated on day t and Dt is the number of days since the experiment began.
Plant hormone measurement
-
Endogenous plant hormones were detected using liquid chromatography-tandem mass spectrometry (LC-MS/MS). Samples were ground into powder in liquid nitrogen and approximately 1 g of the sample was accurately weighed into a test tube. Ten mL of acetonitrile solution and 4 μL of internal standard stock solution were added. The mixture was extracted overnight at 4 °C, then centrifuged at 12,000 g for 5 min at 4 °C, and the supernatant was collected. The precipitate was re-extracted twice with 5 mL of acetonitrile solution, and the supernatants were combined. Impurities were removed by adding an appropriate amount of C18, followed by centrifugation at 12,000 g for 5 min at 4 °C, and the supernatant was collected. The supernatant was then dried under nitrogen gas, redissolved in 200 μL of methanol, filtered through a 0.22 μm organic phase membrane, and stored at −20 °C until analysis. The liquid chromatography conditions were as follows: Column: Poroshell 120 SB-C18 reversed-phase column (2.1 mm × 150 mm, 2.7 μm); Column temperature: 30 °C; Mobile phase: A:B = (methanol/0.1% formic acid) : (water/0.1% formic acid). Elution gradient: flow rate of 0.3 mL/min, 0−1 min: 20%, 1−3 min: 20%−50%, 3−9 min: 50%−80%, 9−10.5 min: 80%, 10.5−10.6 min: decrease to 20%, 10.6−13.5 min: 20%. Mass spectrometry parameters: Ionization mode: ESI in both positive and negative ion modes; Scan type: MRM; Curtain gas: 15 psi; Spray voltage: +4500 V, −4000 V; Nebulizer gas pressure: 65 psi; Auxiliary gas pressure: 70 psi; Nebulizer temperature: 400 °C. Standards for ABA, GA1, GA3, GA4, and GA7 were purchased from Sigma (ABA: Sigma-862169; GA1: Sigma-G7645; GA3: Sigma-G7645; GA4: Sigma-G7276; GA7: Sigma-G7645).
Crude fat and various fatty acid determination
-
Crude fat was measured using the Soxhlet extraction method[19], with each sample analyzed in triplicate. Round-bottom flasks and filter paper thimbles were dried in an oven at 105 ± 2 °C for 2 h, then transferred to a desiccator to cool to a constant weight. The weight of the round-bottom flask was recorded as m0. The filter paper thimble was tared on an analytical balance (accuracy to 0.0001 g), and 3 g of the sample was weighed into the thimble, and recorded as m1. The thimble opening was plugged with defatted cotton. The thimble containing the sample was placed in the extraction tube, and petroleum ether was added to fill two-thirds of the flask. The Soxhlet apparatus was assembled, and cold water was circulated for condensation. The extraction was carried out in a constant temperature water bath at 70−80 °C, allowing the petroleum ether to drip continuously. Extraction continued until no oil traces were detected in the petroleum ether (approximately 6−12 h). After extraction, the thimble was removed with long tweezers and allowed to evaporate the petroleum ether in a well-ventilated area (optimal room temperature: 12−25 °C). The petroleum ether in the extraction flask was recovered. The round-bottom flask was dried at 105 °C for 2 h, cooled in a desiccator, and weighed to obtain m2. Crude fat content was calculated as: Crude fat content = (m2 − m0)/m1 × 100%.
The fatty acid composition of the samples was analyzed using GC-MS. Samples were ground into powder in liquid nitrogen, and 0.5 g of the sample was weighed into a 10 mL centrifuge tube. The sample was soaked in 5 mL of chromatographic methanol for 2 h, then centrifuged at 10,000 rpm for 10 min. The supernatant was extracted twice and combined. The sample was then mixed with 5 mL of chloroform, soaked for 2 h, and centrifuged at 10,000 rpm for 10 min. The supernatant was filtered using a syringe and combined. From each of the two extraction steps, 2.5 mL of the combined supernatant was mixed in a centrifuge tube and dried using a nitrogen evaporator. One mL of 5% potassium hydroxide-methanol solution was added to the dried sample, mixed thoroughly, and heated in a water bath at 60 °C for 30 min. The sample was cooled to room temperature, and 3 mL of 14% boron trifluoride-methanol solution was added. The mixture was heated in a water bath at 60 °C for 3 min and then cooled to room temperature. Finally, 2.5 mL of n-hexane was added, mixed thoroughly, and allowed to stand. The upper clear layer (1.5 mL) was used for GC-MS analysis. GC-MS conditions: Chromatographic column: Agilent DB-225ms, 30 m × 0.25 mm × 0.25 μm; Injection port: 280 °C, split ratio 20:1; Temperature program: initial temperature 50 °C; increase by 5 °C/min to 200 °C, then by 2 °C/min to 230 °C, holding for 10 min; Carrier gas: He, flow rate 1.0 mL/min; Mass spectrometry conditions: ion source temperature 230 °C, quadrupole temperature 150 °C, ionization mode EI, electron energy 70 eV, scan range 35−800 m/z; Injection volume: 1 μL. Fatty acid standards were purchased from Merck (Catalog no. CRM47885).
Gene expression analysis
-
Samples from each treatment were evenly mixed. Approximately 100 mg of each sample was ground to a fine powder in liquid nitrogen, and RNA was extracted using the FastPure Universal Plant Total RNA Isolation Kit (RC411-01, Nanjing, China). RNA concentration and purity were assessed using a NanoDrop spectrophotometer (Thermo Fisher Scientific, Waltham, USA). Total RNA was used for reverse transcription with a High-Capacity cDNA Reverse Transcription Kit (Applied Biosystems, catalog no: 4368814). qPCR reactions were performed on the QuantStudio 5 (Thermo Fisher Scientific, Waltham, USA) using Hieff® qPCR SYBR Green Master Mix (Low Rox Plus) (11202ES03, Yeasen, Shanghai, China). The primers are listed in Supplementary Table S1. The thermal cycling conditions were as follows: initial denaturation at 95 °C for 10 min, followed by 40 cycles of 95 °C for 15 s and 60 °C for 1 min. Melt curve analysis was conducted to verify the specificity of the amplification. Each sample was run in triplicate, and a no-template control was included in each run. Threshold cycle (Ct) values were obtained, and relative gene expression levels were calculated using the 2−ΔΔCᴛ method[20], normalizing to an internal control gene (ELF1B: arahy.E3HYWR).
Yeast one-hybrid assay and dual-luciferase assay
-
Following the instructions provided in the Matchmaker™ Gold Yeast One-Hybrid Library Screening System (Catalog no. 630491, Clontech), the full-length coding sequences (CDS) of three ERF genes were cloned into the pGADT7-AD vector, while the ~2,000 bp promoter regions of two ABI5 genes were cloned into the pAbAi vector. These constructs were subsequently transformed into Y1H yeast strains. The interactions were assessed on a selective medium lacking leucine and supplemented with 800 ng/mL aureobasidin A. Detailed information on the primers used for yeast one-hybrid vector construction is provided in Supplementary Table S1.
The full-length CDS of three ERF genes was cloned into the pGreenII 0029 62-SK vector, while the ~2,000 bp promoter sequences of two ABI5 genes and one ACO gene were cloned into the pGreenII 0800-LUC vector. Empty and recombinant vectors were introduced into Agrobacterium tumefaciens strain GV3101-p19 and subsequently infiltrated into Nicotiana benthamiana leaves. After 48 h, fluorescence was detected using an in vivo imaging system (NightShade LB985, Berthold, Germany), and the LUC/REN ratio was determined using a luciferase assay kit (Promega, Madison, WI, USA). The primers used are listed in Supplementary Table S1.
Statistical analysis
-
Statistical analysis was performed using GraphPad Prism. To compare the means of the two groups, a T-test was used to assess the significance of differences between treatments at the same time point. For comparisons involving more than two groups, ANOVA was employed to assess the significance of differences between treatments at the same time point. Significance levels were denoted as * for p-value ≤ 0.05 and for ** p-value ≤ 0.01.
-
Given the limited research on the role of ethylene in promoting peanut seed germination, we initially employed four varying concentrations: 0.5, 1.0, 2.0, and 5.0 mM, to treat the peanut seeds. We utilized two different treatment methods: continuous soaking in a fixed ethylene concentration for 7 d; or soaking in a fixed ethylene concentration for 12 h followed by sterile water soaking. The results indicated that high ethylene concentrations of 5.0 mM inhibited peanut seed germination, whether the seeds were continuously soaked in ethylene or initially soaked in ethylene followed by sterile water (Supplementary Fig. S1). For both treatment methods and concentrations, no significant differences were observed between these concentration gradients and the control. Soaking in 0.5 mM ethylene resulted in higher germination rates ((Number of germinated seeds/Total number of seeds tested) × 100%) on the 2nd, 3rd, and 7th d (Supplementary Fig. S1a), along with a higher germination index (Germination index = ∑(Gt/Dt), where Gt is the number of seeds germinated on day t and Dt is the number of days since the experiment began) (Supplementary Fig. S1b), although the differences were not statistically significantly. However, after soaking in 0.5 mM ethylene for 12 h followed by water soaking, a higher germination rate was observed only on the second day compared to other treatments (Supplementary Fig. S1c). At other times, the germination rate was lower than the control, and the germination index was also below that of the control (Supplementary Fig. S1d).
0.2 mM ethylene promotes peanut seed germination
-
Given the inhibitory effect of high ethylene concentrations on germination, we tested the influence of lower concentrations (0.1, 0.2, and 0.4 mM) on seed germination. We found that both continuous ethylene treatment and soaking in ethylene for 12 h followed by water soaking showed that 0.2 mM ethylene significantly enhanced the germination rate and the proportion of seeds with radicles longer than 3 mm (Supplementary Fig. S2). Compared to continuous ethylene treatment, 12-h ethylene soaking followed by water soaking resulted in a higher final germination rate and a higher percentage of seeds with radicles exceeding 3 mm (Supplementary Fig. S2c & d). This may be due to the continuous ethylene treatment leading to ethylene buildup, which could inhibit further seed germination. The 0.2 mM ethylene + H2O treatment exhibited higher germination rates at both 12 and 24 h (Supplementary Fig. S2e), leading us to choose this treatment for further study.
Compared to the control, the 0.2 mM ethylene + H2O treatment significantly increased the germination rate within the first 3 d (Fig. 1a). Specifically, the germination rate exceeded 80% under ethylene treatment by 24 h, compared to less than 60% in the control group, highlighting ethylene's role in accelerating the germination process. The radicle growth rate was notably faster at 72 h (third day) than in the control (Fig. 1b & d). The germination index, which considers both the speed and success of germination by accounting for the number of seeds germinated and the time taken, was significantly higher with the 0.2 mM ethylene + H2O treatment than in the control. The index value for the ethylene treatment was over 20% higher than that of the control, which was below 20%, indicating that ethylene enhanced both the rate and uniformity of seed germination (Fig.1c).
Figure 1.
0.2 mM ethylene + H2O enhances peanut seed germination, radicle growth, and gibberellin synthesis. (a) Comparison of germination rates between the control and 0.2 mM Ethylene + H2O treatment. (b) Percentage of seeds with radicles exceeding 3 mm in the control and 0.2 mM Ethylene + H2O treatment. (c) Comparison of germination indexs between the control and 0.2 mM Ethylene + H2O treatment. (d) Radicle growth at 72 h (third day) between the control and 0.2 mM Ethylene + H2O treatment. (e) Concentrations of abscisic acid and gibberellins in the control compared to the 0.2 mM Ethylene + H2O treated samples. *, p-value ≤ 0.05, **, p-value ≤ 0.01.
Ethylene treatment enhances gibberellin concentration
-
Abscisic acid (ABA) maintains seed dormancy and prevents early germination, while gibberellins (GAs) are essential for breaking dormancy. The interaction between ABA and GAs crucially regulates dormancy and germination[21]. Additionally, studies have shown that ethylene interacts with ABA and GA pathways, countering the effects of ABA by modifying its metabolism and signaling[22,23]. Therefore, the concentrations of abscisic acid (ABA) and gibberellins (GAs) in the germinating seeds were tested. The results indicated that during the first 12 h of germination, ABA levels initially increased and then decreased, with no significant difference between the ethylene-treated and control groups. The ABA concentration peaked at 4 h in both groups, exceeding 10 ng/g, before decreasing to less than 10 ng/g by 12 h, highlighting its transient role in the early stages of germination. In contrast, the levels of GA1, 3, and 4 consistently increased during the first 12 h of germination. Ethylene treatment significantly enhanced the increase of GA1, 3, and 4, particularly at 4 and 8 h, notably boosting GA3 and GA4 levels. For GA7, there was an initial increase followed by a decrease within the first 12 h; ethylene significantly promoted the rise of GA7 at 4 h, but by 8 h, it significantly reduced GA7 levels. Thus, hormone assays revealed that ethylene mainly enhanced the upregulation of GA3 and GA4 (Fig. 1e).
The observed changes in GA and ABA levels reflect their distinct roles in regulating seed germination. The transient increase in ABA levels at the early stage of germination may act as a checkpoint, ensuring seeds germinate only under favorable conditions. However, the subsequent decline in ABA levels alleviates its inhibitory effect on germination. The consistent increase in GA levels, especially GA3 and GA4, is critical for promoting seed germination by stimulating growth processes, weakening the seed coat, and activating enzymes necessary for radicle emergence. Ethylene's enhancement of GA3 and GA4 levels highlights its role in shifting the hormonal balance toward germination, thereby breaking dormancy and facilitating seedling development under optimal conditions.
Ethylene treatment disrupted the expression of its own and related genes involved in ABA and GA pathways
-
Considering the crosstalk among ethylene, ABA, and GA, we further analyzed the expression changes of key genes involved in the synthesis, degradation, and signaling of these hormones. These genes, highly expressed in seeds, were identified in the study by Clevenger et al.[24] (Supplementary Table S2). During seed germination, the endogenous ethylene synthesis-related genes ACS (1-Aminocyclopropane-1-Carboxylate Synthase) and ACO (1-Aminocyclopropane-1-Carboxylate Oxidase) were significantly upregulated, with the rate-limiting enzyme ACO showing a notable increase: compared to 0 h, ACO expression increased by over 500 times in the control and over 800 times under ethylene treatment at 12 h, indicating that ethylene treatment significantly boosted ACO expression (Fig. 2). Additionally, the ethylene receptor gene ETR1 (Ethylene Response Sensor) also showed significant upregulation during germination: increasing by over 15 times in the control and over 30 times under ethylene treatment at 12 h, similarly to ACO, ethylene treatment significantly promoted ETR1 expression.
Figure 2.
Gene expression changes of key genes involved in the synthesis, degradation, and signaling of ethylene, ABA, and GA. During the first 12 h of germination, genes exhibiting more than a tenfold change in expression are highlighted with a darker background. *, p-value ≤ 0.05, **, p-value ≤ 0.01. ACS: 1-Aminocyclopropane-1-Carboxylate Synthase; ACO:1-Aminocyclopropane-1-Carboxylate Oxidase; ETR1: Ethylene Response Sensor 1; CTR1: Constitutive Triple Response 1; EIN2: Ethylene Insensitive 2; EIN3: Ethylene Insensitive 3; ERF1B: Ethylene Response Factor 1B; ABA1: Zeaxanthin Epoxidase; NCED: 9-cis-epoxycarotenoid dioxygenase; AAO3: Abscisic Aldehyde Oxidase 3; CYP707A: Cytochrome P450, Family 707, Subfamily A; ABI5: Abscisic Acid Insensitive 5; PP2C: Protein Phosphatase 2C; GA20ox: Gibberellin 20 Oxidase; GA2ox: Gibberellin 2 Oxidase; GAMT: Gibberellin Methyltransferase; GID: Gibberellin Insensitive Dwarf; DELLA: Aspartic acid (D), Glutamic acid (E), Leucine (L), Leucine (L), and Alanine (A)—in a conserved region of the protein; SLY1: SLEEPY1.
During seed germination, three ABA synthesis-related genes and two ABI5 (Abscisic Acid Insensitive 5) genes were downregulated. However, no significant differences were observed between the control and ethylene treatments at 4, 8, and 12 h. Interestingly, the expression of two ABI5 was significantly inhibited after 12 h of ethylene treatment (corresponding to 0 h of germination). Two genes regulating ABA degradation, CYP707A (Cytochrome P450, Family 707, Subfamily A), showed increased expression during seed germination. The trends differed between ethylene treatment and control: one gene's expression was significantly reduced, while the other's expression was significantly increased under ethylene treatment. Additionally, there was no significant differences in the expression of the ABA signaling-related PP2C (Protein Phosphatase 2C) gene between ethylene and control (Fig. 2). In summary, the rapid inhibition of ABA insensitive gene in response to ethylene treatment begins before seed germination. Therefore, ethylene may promote seed germination by interfering with ABA signal transduction through the inhibition of ABI5 gene expression. The downregulation of ABA synthesis-related genes and receptors during seed germination aligns with previous studies indicating ABA's negative regulation of seed germination[25,26].
Regarding GA-related genes, the expression pattern showed that GA20ox (Gibberellin 20 Oxidase), associated with GA synthesis, decreased during seed germination, while GA2ox (Gibberellin 2 Oxidase), associated with GA metabolism, increased. However, no significant trend of difference was observed between ethylene treatment and control. Among the evaluated gibberellin-related genes, the expression of one of the GID (Gibberellin Insensitive Dwarf) genes, arahy.ELEE28, significantly increased during germination. Specifically, its expression rose approximately 6-fold in the control and 9-fold under ethylene treatment at 12 h compared to 0 h, demonstrating that ethylene significantly boosts the expression of GID-arahy.ELEE28 at 12 h (Fig. 2). Although previous hormone assays indicated that ethylene treatment increased GA levels in germinating seeds, no clear trends were observed in the expression of GA synthesis-related genes, possibly due to the complex nature and multiple active forms of GA involved in plants.
Considering the inhibitory effect of ABI5 on seed germination[27], we hypothesized that ethylene treatment suppresses the expression of ABI5, thereby promoting seed germination. To test this hypothesis, we constructed two ABI5 promoter-luciferase reporter vectors, transformed them into Agrobacterium, and injected them into tobacco leaves followed by ethylene application. Given that exogenous ethylene can activate its synthesis[28], our qPCR results also confirmed this effect, ethylene treatment increased the expression of ACO gene (Fig. 2). Therefore, we used the ACO gene promoter as a control. Using a luciferase assay, we confirmed that ethylene indeed activated ACO expression while suppressing ABI5 expression (Fig. 3a).
Figure 3.
Ethylene inhibits ABI5 expression through ERFs. (a) Dual-luciferase assays confirmed that ethylene activated ACO expression while suppressing ABI5 expression. (b) Gene expression changes of three ERF genes. Two of them (arahy.HGAZ7D and arahy.QGFJ76) contained repressor motifs. (c) Yeast one-hybrid assays confirmed that all three ERFs bound to the promoter of ABI5. (d) Dual-luciferase assays confirmed that all three ERFs inhibited ABI5 expression. *, p-value ≤ 0.05; **, p-value ≤ 0.01.
Ethylene typically regulates the expression of other genes by influencing ethylene response factors (ERFs). Through signal transduction, ethylene activates ERFs, which subsequently regulate downstream gene expression either directly or indirectly[28]. Based on previous studies, we selected three ERFs (arahy.HGAZ7D, arahy.QGFJ76, and arahy.ZW7540) highly expressed in seeds, two of which (arahy.HGAZ7D and arahy.QGFJ76) contain EAR repressor domains[29] (Fig. 3b). We analyzed their response to ethylene treatment and their role in regulating ABI5. qPCR analysis revealed that all three ERFs exhibited varying levels of upregulation upon ethylene treatment. Specifically, arahy.QGFJ76 showed significant upregulation at 0 and 4 h, arahy.ZW7540 exhibited significant upregulation at 4, 8, and 12 h, and while arahy.HGAZ7D also displayed upregulation at 4, 8, and 12 h, these changes were not statistically significant. Furthermore, we performed yeast one-hybrid assays to determine whether these transcription factors could bind to the ABI5 promoter. The results demonstrated that all three ERFs bind to the ABI5 promoter (Fig. 3c). Dual-luciferase assays further confirmed that all three ERFs negatively regulate ABI5 expression (Fig. 3d). In conclusion, ethylene promotes the expression of ERFs, which in turn suppress ABI5 expression, thereby facilitating seed germination.
Ethylene treatment enhances fatty acid degradation
-
As is well known, peanuts are an important oil crop, with the oil content in their seeds reaching about 50%[30]. Therefore, we wanted to understand how the fatty acid composition changes during peanut germination, and whether ethylene affects this process. Consequently, we measured the fatty acid content in seeds treated with ethylene and control seeds over a period from 0 to 12 h.
Firstly, we measured the changes in total crude fat content and found that although there was an overall reduction within 12 h of germination, the decrease was not significant. However, ethylene treatment significantly accelerated the reduction of crude fat at 8 and 12 h (Fig. 4a). Further, we analyzed the changes in different fatty acid components in the samples using GC-MS (Gas Chromatography-Mass Spectrometry). The results showed that the three most abundant fatty acids in peanut seeds were 9,12-Octadecadienoic acid (C18:2), 9-Octadecenoic acid (C18:1), and Hexadecanoic acid (C16:0), which accounted for approximately 37.86%, 28.98%, and 13.57% of the total detected fatty acids, respectively (Supplementary Fig. S3), similar to results found in previous studies[31,32]. The levels of all 18 detected fatty acids decreased during seed germination. Compared to the control, ethylene treatment variably accelerated the degradation of all fatty acids except for cis-11,14-Eicosadienoic acid (C20:2), Heneicosanoic acid, methyl ester (C21:0), and Hexacosanoic acid, methyl ester (C26:0) (Fig. 4b; Supplementary Fig. S4). Notably, the highest content of 9,12-Octadecadienoic acid and 9-Octadecenoic acid showed significant reduction at 8 h (Supplementary Fig. S4).
Figure 4.
Ethylene enhances fatty acid degradation during seed germination. (a) Change in crude fat concentration between the control and 0.2 mM Ethylene + H2O treatment during germination. (b) GC-MS (Gas Chromatography-Mass Spectrometry) analysis of fatty acid composition changes in samples between control and ethylene treatment, the detailed data can be found in Supplementary Table S3 and Supplementary Fig. S3. (c) Expression changes of key genes in the beta-oxidation pathway during seed germination, comparing control and ethylene treatment. *, p-value ≤ 0.05; **, p-value ≤ 0.01. CTS: Comatose/Peroxisomal ABC Transporter; ACS#: Acyl-CoA Synthetase. #, not to be confused with the ethylene-related ACS; MFP: Multifunctional Protein–Contains 2E-enoyl-CoA hydratase and 3S-hydroxyacyl-CoA dehydrogenase activities; KAT: 3-Ketothiolase.
Beta-oxidation is the principal pathway implicated in fatty acid degradation in the peroxisomes of plants[33]. Therefore, we examined the expression changes of key enzymes in the beta-oxidation pathway during seed germination (Fig. 4c). We found that genes located upstream and downstream of beta-oxidation, CTS (Comatose/Peroxisomal ABC tranporter) and KAT (3-ketothiolase), were upregulated during seed germination. Notably, KAT-arahy.K55J2B was upregulated nearly 9-fold at 12 h compared to 0 h, suggesting it may be one of the key enzymes in fatty acid degradation during seed germination. However, compared to the control, ethylene did not significantly enhance the upregulation of these genes, except KAT-arahy.74EXT1, which was significantly higher at 12 h (Fig. 4c).
Ethylene treatment enhances the expression of cell wall-loosening related genes
-
In a transcriptome comparison of different peanut seed varieties during germination, Li et al. identified several differentially expressed genes related to the cell wall[34]. We selected four significantly different genes for validation in our material: EXP (Expansin, LOC112698064/arahy.0QA3UW), PEC (Pectinesterase, LOC112695783/arahy.SBT9R0), and two ARAB genes (arabinogalactan protein, LOC112784877/arahy.3QT14C and LOC112769149/arahy.D2IUDG). The results showed that two genes, EXP and PEC, were significantly upregulated during seed germination, and their expression was significantly enhanced by ethylene treatment, consistent with previous findings. Although ARAB-arahy.3QT14C was not upregulated during germination, ethylene still promoted its expression. This variation might be due to the presence of multiple homologous ARAB genes, with differentially expressed genes responding to different treatments or varying across different varieties (Fig. 5).
-
Ethylene regulates various aspects of plant growth and development, including agriculturally important processes such as seed germination and fruit ripening[14]. Ethylene promotes seed germination in various plants, as demonstrated in Arabidopsis[17], wheat[35], soybean[36], and sunflower[37]. While ethephon treatment is an effective method for breaking peanut seed dormancy[11], the specific and complete mechanism is not yet perfect. The mechanism by which ethylene facilitates seed germination involves several physiological and biochemical processes. Ethylene is known to enhance the activity cell wall-related enzymes, which degrade the cell wall components of the endosperm, making it easier for the radicle to emerge[38]. Additionally, ethylene modulates the expression of genes involved in cell wall loosening and expansion, further aiding the germination process[39]. Ethylene also influences the synthesis and signaling of other hormones like gibberellins (GA) and abscisic acid (ABA), which are crucial for seed germination. It has been observed that ethylene can reduce the levels of ABA, a hormone that inhibits germination while promoting the synthesis of GA, which stimulates the germination process[22]. This dual action helps to create a hormonal environment conducive to seed germination.
Ethylene does not act in isolation, it interacts with other plant hormones to regulate seed germination. The crosstalk between ethylene and other hormones like GAs, ABA, and auxins plays a significant role in determining the outcome of seed germination. Ethylene promotes the biosynthesis of GA, which is essential for breaking seed dormancy and promoting germination. GA stimulates the production of hydrolytic enzymes that degrade the seed coat and endosperm, facilitating radicle emergence[40]. The interaction between ethylene and GA is synergistic, with ethylene enhancing the sensitivity of seeds to GA. ABA is a key inhibitor of seed germination. Ethylene reduces ABA levels by promoting its catabolism through the upregulation of ABA 8'-hydroxylase genes (CYP707A)[41]. This reduction in ABA levels alleviates its inhibitory effects, thus promoting seed germination. Ethylene also interacts with auxins to regulate seed germination and early seedling growth. Auxins are involved in cell elongation and division, processes that are essential for the emergence of the radicle. Ethylene can modulate the distribution and transport of auxins, thereby influencing seed germination[42].
In our study, we found that ethylene promotes seed germination by increasing GA levels (primarily GA3 and GA4), inducing endogenous ethylene synthesis, enhancing ethylene signal transduction, interfering with ABA signal transduction, boosting GA signal transduction, promoting fatty acid degradation, and facilitating cell wall loosening. Notably, the ethylene synthesis rate-limiting gene ACO, the ethylene receptor ETR, and the cell wall loosening genes EXP and PEC were significantly upregulated by ethylene treatment. In contrast, the abscisic acid-insensitive gene ABI5 was significantly downregulated, as ethylene promotes the expression of ERFs, which in turn suppress ABI5 expression (Fig. 6). Thus, our study demonstrated that in peanuts, ethylene promotes seed germination through a mechanism similar to that observed in other species. This mechanism involves balancing hormones such as ABA and GA, as well as modulating the activity of cell wall-related enzymes.
Figure 6.
Schematic depiction of ethylene's effect on peanut seed germination. Round rectangles represent genes, ovals represent plant hormones, and shapes with sharp corners represent fatty acid. EXP: Expansin; PEC: Pectinesterase; ABI5: Abscisic Acid Insensitive 5; GID: Gibberellin Insensitive Dwarf; ACO: 1-Aminocyclopropane-1-Carboxylate Oxidase; ETR: Ethylene Response Sensor; ERF: Ethylene Response factor.
Ethylene promotes the germination of seeds in various species, with its effectiveness depending on the species and the depth of dormancy. There is a specific range of effective ethylene concentrations for promoting seed germination. In our study, we found that high concentrations of ethylene inhibited peanut seed germination, while the optimal concentration range was 0.2−0.4 mM (Fig. 1; Supplementary Fig. S2). Previous research indicated that fumigating Virginia-type peanut seeds with an external concentration of 3.0 to 3.5 ppm (equivalent to 0.12−0.13 mM) for 6 h was sufficient to break dormancy[43], which aligns with our findings. Both our study and previous research suggest that continuous application of ethylene is not necessary; a brief treatment with an appropriate concentration is sufficient to promote seed germination. Our research shows that regardless of ethylene treatment, peanut seed germination is accompanied by a rapid increase in the expression of the rate-limiting enzyme-ACO for endogenous ethylene synthesis, and exogenous ethylene can significantly enhance ACO expression. During pea seed germination, ethylene also promotes its biosynthesis through a positive feedback regulation of ACO[44]. Additionally, studies on pea and wheat have shown that ethylene production starts early after imbibition and reaches a maximum before mitotic activity[45]. In non-dormant lettuce seeds, ethylene production increases rapidly during germination, peaking at 24 h, which coincides with the emergence of the radicle and then declines[46]. These findings indicate that ethylene is an essential hormone involved in seed germination.
In cruciferous seeds, ethylene promotes the weakening and rupture of the endosperm cap, counteracting the inhibitory effects of ABA on these processes, thereby facilitating radicle emergence[17]. ABA inhibits the activity and expression of ACC oxidase (ACO), a key enzyme in ethylene biosynthesis[22,47]. The impact of increased ethylene levels on ABA content varies across different studies. For instance, in the ethylene-overproducing mutant eto1-1, elevated ethylene levels lead to increased ABA content[48]. However, treatment with exogenous ethylene or its precursor ACC does not affect ABA content or the expression of genes involved in ABA biosynthesis in Lepidium sativum[17], and sugar beet[49]. Some studies suggest that high concentrations of ABA and ethylene mutually inhibit each other, while low concentrations may activate each other's biosynthesis under certain environmental conditions[50]. However, the mechanisms by which they inhibit or specifically stimulate each other at the transcriptional level, or whether they interact or operate in parallel, require further investigation.
In our experiment, exogenous ethylene treatment elevated ABA content, though not significantly compared to the control. However, it significantly inhibited the expression of two ABI5 genes after 12 h of ethylene treatment (corresponding to 0 h of germination) (Fig. 2). In Arabidopsis, ethylene was found to inhibit the expression of ABA-responsive genes and reduce ABA sensitivity[51]. Additionally, the application of ethylene or its precursor ACC led to a reduction in ABA levels or their signaling components, thereby alleviating ABA-mediated dormancy[17]. In our study, ethylene activates the ERF genes, and then these transcriptors directly inhibit the expression of abscisic acid-insensitive ABI5, and inhibited the expression of them (Fig. 3). Previous reports have also shown that certain ERF transcription factors can bind to the ABI5 promoter and inhibit its expression. For example, in Arabidopsis, RAV1, a type of ERF, has been found to associate with the promoters of ABI3, ABI4, and ABI5 both in vitro and in vivo, suggesting that RAV1 directly represses the transcription of these genes[52].
The findings of this study have significant implications for agricultural practices, particularly in enhancing the germination efficiency of peanut seeds and other crops with similar dormancy mechanisms. The ability of ethylene to modulate hormonal pathways, such as reducing ABA levels and enhancing GA synthesis, provides a targeted approach to breaking dormancy and promoting uniform germination. The identification of optimal ethylene concentrations (0.2–0.4 mM) is especially valuable, as it minimizes the risk of inhibitory effects associated with excessive ethylene levels. This insight can guide the development of cost-effective treatments, such as short-term ethylene fumigation or soaking in ethylene-releasing compounds, to achieve faster and more uniform germination. These methods can be particularly beneficial in commercial seed production, where rapid and consistent germination is critical for crop establishment and yield. Furthermore, understanding ethylene's interaction with ABA and GA pathways opens new possibilities for integrating hormonal treatments with other dormancy-breaking strategies, such as temperature adjustments or seed priming, to further enhance agricultural efficiency and adaptability to various environmental conditions. These applications could significantly improve germination success rates, particularly in regions where environmental constraints challenge seed viability and crop productivity.
-
Our study reveals that ethylene significantly enhances 'HuaGuan' peanut seed germination by increasing gibberellin levels, inducing ethylene synthesis and signaling, and disrupting abscisic acid signaling. Key genes involved in ethylene synthesis, signaling, and cell wall loosening were upregulated, while the ABA-insensitive gene was downregulated. Furthermore, it was confirmed that ethylene enhances the expression of three ERFs, which bind to the ABI5 promoter and inhibit its expression, thereby promoting seed germination. Notably, ethylene also promoted fatty acid degradation, a novel finding in peanut seeds. These insights into ethylene's role in hormonal modulation and metabolic adjustments offer practical applications for improving seed germination practices in agriculture.
This work was supported by the Taishan Scholars Program of Shandong Province (tsqn202103161), the Key R&D Program of Shandong Province, China (2024LZGC035), the Weifang Science and technology development plan (2024JZ001), and the Natural Science Foundation of Shandong Province (ZR202103010405) to Xiaoqin Liu. It was also founded by the Taishan Scholar Project Funding, China Agriculture Research System (Project No. CARS-13) to Xiaoyuan Chi.
-
The authors confirm contribution to the paper as follows: funding support, study conception and design: Liu X; material treatment, data analysis and manuscript writing: Cui Y, Guo H, Wang Q, Meng Q, Li T; material preparing: Zhang J, Chi X, Fu C, Cui F. All authors reviewed the results and approved the final version of the manuscript.
-
The datasets generated during and/or analyzed during the current study are available from the corresponding author on reasonable request.
-
The authors declare that they have no conflict of interest.
-
# Authors contributed equally: Yuanyuan Cui, Haosong Guo
- Supplementary Table S1 Primers used in this study.
- Supplementary Table S2 Expression levels of key genes involved in the synthesis, degradation, and signaling of plant hormones in different tissues, based on Clevenger et al.[24].
- Supplementary Table S3 Fatty acid content in various components, supplementing Fig. 4b. *, p-value ≤ 0.05; **, p-value ≤ 0.01.
- Supplementary Fig. S1 The effects of varying ethylene concentrations (0.5 mM, 1.0 mM, 2.0 mM, and 5.0 mM) on the germination rate and germination index of peanut seeds using different treatment methods: continuous ethylene soaking, or ethylene soaking for 12 hours followed by sterile water soaking.
- Supplementary Fig. S2 The influence of lower concentrations (0.1, 0.2, and 0.4 mM) on seed germination.
- Supplementary Fig. S3 Proportions of different fatty acids in peanut seeds.
- Supplementary Fig. S4 Bar chart showing differences in various fatty acid components between treatment and control groups, supplementing Fig. 4b. *, p-value ≤ 0.05; **, p-value ≤ 0.01.
- Copyright: © 2025 by the author(s). Published by Maximum Academic Press on behalf of Hainan Yazhou Bay Seed Laboratory. This article is an open access article distributed under Creative Commons Attribution License (CC BY 4.0), visit https://creativecommons.org/licenses/by/4.0/.
-
About this article
Cite this article
Cui Y, Guo H, Wang Q, Meng Q, Li T, et al. 2025. Ethylene enhances peanut seed germination by modulating hormonal and metabolic pathways. Seed Biology 4: e005 doi: 10.48130/seedbio-0025-0005
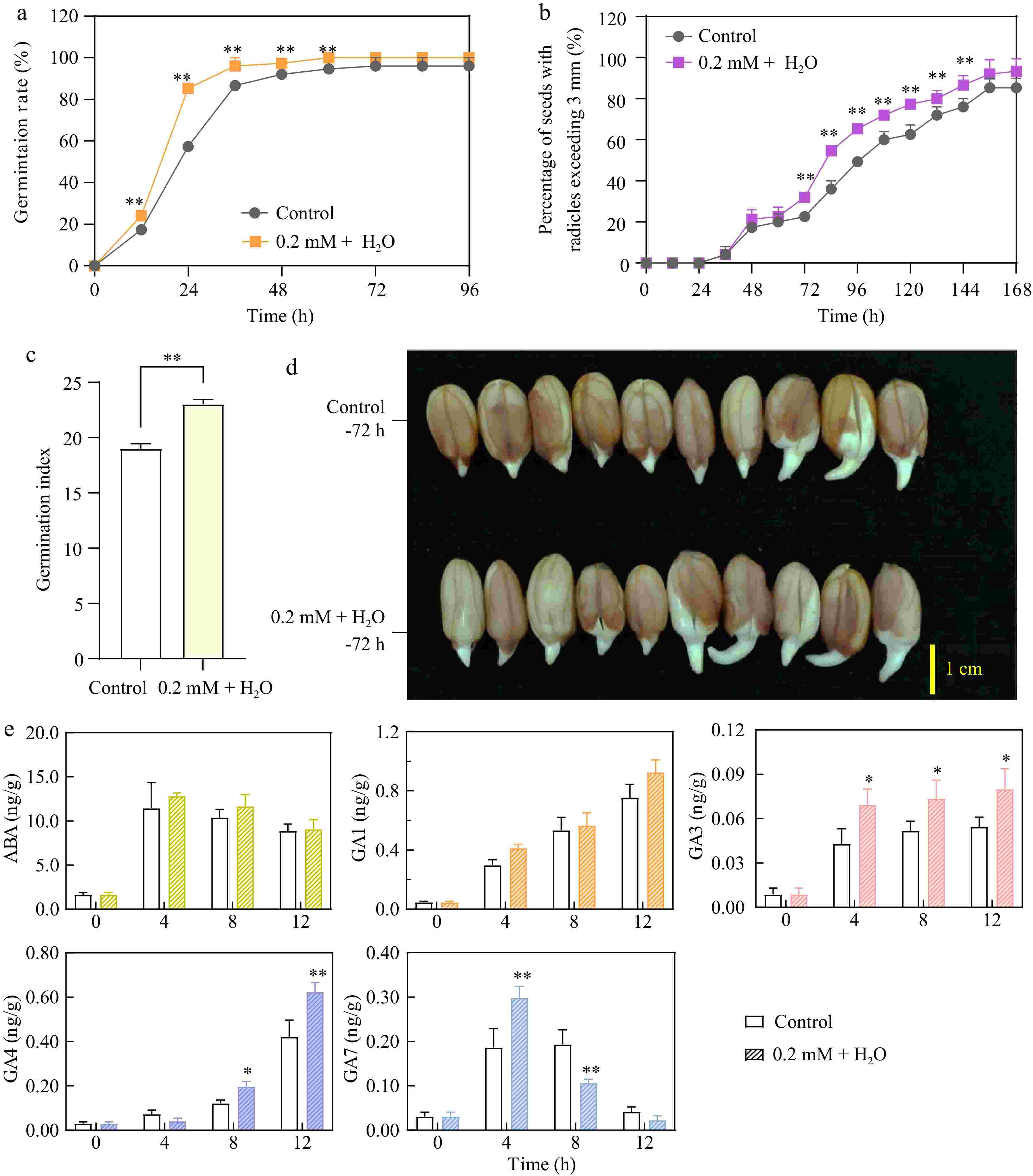