-
Plastics have become an indispensable part of modern society, thanks to their light weight, low cost, durability, and the versatility to be tailored for various applications. Over the past few decades, global plastic production has grown exponentially, reaching approximately 400 million tons in 2022, which has resulted in a substantial accumulation of waste plastics[1,2]. It is well-known that the degradation of waste plastics under natural conditions can take hundreds, or even thousands of years. The massive quantity of waste plastics has placed a significant strain on ecosystems and has led to severe environmental issues. Moreover, waste plastics in the natural environment break down into microplastics that can enter the human body through the food chain, posing a serious threat to human health[3]. As the issue of 'white pollution' intensifies, there is growing recognition of the severity and urgency of addressing waste plastics, prompting increased efforts to find viable solutions to plastic pollution.
Landfill and incineration are two common methods for disposing of waste plastics, accounting for approximately 72% and 19%, respectively[1]. However, both have significant drawbacks. Landfills consume valuable land resources, and harmful substances in waste plastics can easily contaminate the air, soil, and groundwater[4,5]. While incineration generates energy that can be used for electricity and heat, the combustion process releases large amounts of smoke, solid particles, and hazardous substances, such as furans, dioxins, and polychlorinated biphenyls[6]. To mitigate plastic pollution and promote the reuse of waste plastics, several recycling methods have been proposed, including mechanical recycling, bio-recycling, and chemical recycling[7]. Mechanical recycling is the only widely implemented method for large-scale waste plastic disposal, involving processes such as collecting, sorting, cleaning, shredding, and melt-reprocessing[8]. However, it is primarily limited to polyethylene terephthalate (PET) and polyethylene (PE), and is not suitable for temperature-sensitive plastics (e.g., polyvinyl chloride (PVC)) or plastics that don't flow at elevated temperatures[9,10]. Additionally, each step in the mechanical recycling process leads to a degradation of the mechanical properties[8,10]. Bio-recycling is a promising recycling method that converts plastics into valuable products through microbial and enzymatic degradation[11]. However, the development of this technology is still limited due to low yields and high downstream costs[12].
Chemical recycling refers to the chemical conversion of waste plastics into short-chain organic molecules or monomers that can be used as feedstocks to manufacture new materials, mainly including hydrogenolysis[13], pyrolysis[14], solvolysis[15], and photocatalysis[16]. Compared to mechanical recycling and bio-recycling, chemical recycling can process a wider range of plastics[17]. Among these methods, photocatalytic technology is particularly promising due to its use of sunlight, mild reaction conditions, efficient processing, sustainability, and environmental friendliness[18]. Currently, photocatalytic degradation and conversion are the two primary research focuses in the photocatalytic treatment and disposal of waste plastics. Photocatalytic degradation aims to break down plastics into environmentally benign small molecules (CO2, H2O, etc.), while photocatalytic conversion seeks to extract value-added products from the abundant hydrocarbon resources in plastics[19,20]. Additionally, photocatalytic hydrogen production is a key research direction, focusing on the synergy between plastic oxidation and hydrogen ion (H+) reduction[21].
This review aims to provide an overview of recent studies on the application of photocatalytic technology in the treatment and disposal of plastics, as shown in Fig. 1. Firstly, the various types of photocatalysts used for plastic disposal and their roles in the reaction processes are introduced. Next, recent advances in photocatalytic degradation and conversion are discussed, along with the synergy between plastic oxidation and hydrogen production. Then, key factors influencing photocatalytic reactions are summarized. Finally, this review outlines the challenges and future directions for the development of photocatalytic technology in this field. It's hoped that this article will inspire researchers to conduct follow-up studies.
-
Photocatalysts play an important role in the photocatalytic reaction process. When a photocatalyst absorbs photons with energy greater than its band gap, it generates photogenerated carriers. These carriers then migrate to the surface of the photocatalyst, where they react with the reactants[22−24]. The efficiency of the photocatalytic reaction largely depends on the selection and design of the photocatalysts. Currently, photocatalysts can be classified into three categories based on their development: bare photocatalysts, modified photocatalysts, and some novel photocatalysts, as shown in Fig. 2. Bare photocatalysts, the earliest used in photocatalytic reactions, are typically made from raw materials without any physical or chemical treatment. Their photocatalytic performance is primarily determined by their inherent optical absorption properties and energy band structures. However, bare photocatalysts generally suffer from limited sunlight absorption, high recombination of electron-hole pairs, and low photocatalytic activity. As the understanding of the photocatalytic mechanisms deepens, researchers have begun modifying bare photocatalysts through methods such as elemental doping and heterostructure fabrication. In recent years, significant progress has been made in the development of novel photocatalysts, driven by the emergence of new materials and technologies. These novel photocatalysts show great potential and are expected to play a key role in future applications.
Bare photocatalysts
-
Bare photocatalysts can be classified into metal photocatalysts and non-metal photocatalysts. Metal photocatalysts are primarily composed of metal oxides and sulfides, with key metal elements including titanium (Ti)[23], cadmium (Cd)[22], zinc (Zn)[24], bismuth (Bi)[28],
niobium (Nb)[29], copper (Cu)[30], and iron (Fe)[25].Based on the size of their band gap, metal oxide photocatalysts are classified into wide-band (> 2.3 eV) and narrow-band catalysts (Fig. 3). Wide-band metal oxide photocatalysts, such as titanium dioxide (TiO2) and zinc oxide (ZnO), exhibit excellent redox capacities and generate high-energy photogenerated carriers, making them suitable for catalyzing plastics with strong C-C bonds, such as polyolefins and PVC. For example, TiO2 (Eg = 3.2 eV), an N-type semiconductor material, is one of the most commonly used metal oxide photocatalysts due to its high stability, nontoxicity, low cost, photo corrosion resistance, and great oxidation capacity[31]. Besides, ZnO (Eg = 3.2 eV) is considered a strong competitor to TiO2 because it is cheaper and easier to synthesize. It also has low toxicity, good oxidation capacity, and favorable structural properties that enhance electron mobility[32]. However, the wide-band gaps of these catalysts absorb ultraviolet (UV) light, limiting their large-scale industrial application[33]. In contrast, narrow-band metal oxide photocatalysts can utilize visible light for photocatalytic reactions while maintaining strong oxidation capacities. For example, copper oxides (CuO, Cu2O, etc.) have lower band gaps than TiO2, enabling them to generate high-energy photogenerated holes (h+) under visible light irradiation without adding dopants[30]. However, the photogenerated electrons (e-) produced by these catalysts usually have low energy, which limits their reduction reactions with H2O/H+/O2.
Metal sulfides generally have weaker oxidation capacities compared to metal oxides, making them more suitable for catalyzing short-chain plastics and small molecules after plastic pretreatment[35,36]. Currently, most metal sulfide photocatalysts used for plastic disposal contain the element Cd[22,36,37]. Among various metal sulfides, CdS stands out as one of the most prominent visible-light-responsive photocatalysts due to its excellent reduction capacity, strong light absorption, and simple preparation methods[38,39]. However, Cd-based catalysts are toxic, and their poor photochemical stability limits their ability to participate continuously in photocatalytic reactions over extended periods.
All non-metal photocatalysts currently used for plastic disposal are carbon nitrides[40,41], such as graphitic-phase carbon nitride (g-C3N4) and graphene oxide (GO). g-C3N4 has become a research focus in this field due to its strong light absorption, great photochemical stability, and moderate oxidation capacity[42]. It is also abundant on Earth and more environmentally friendly than metal photocatalysts. Besides, GO is considered an outstanding catalyst promoter because of its hydrophilic nature, large specific surface, and high optical transmittance[43,44].
Modified photocatalysts
-
Bare photocatalysts generally suffer from limited sunlight absorption, high recombination of electron-hole pairs, and low photocatalytic activity. To obtain suitable energy band structures, promote the separation and transfer of photogenerated carriers, and improve interfacial photocatalytic performance, modifications such as elementary doping, heterojunction construction, and electrochemical method are necessary[24,26] (Table 1).
Table 1. Modified photocatalysts and their characteristics.
Photocatalysts Preparation methods Band gap
(eV)Light absorption (nm) Improvement Application Ref. Elementary doping Au/TiO2 Chemical deposition 2.40−2.98 586 Surface defects Hydrogen production of PET [45] F-TiO2 Immersion method 1.50 / Photogenerated carrier separation Degradation of PE [23] N-TiO2 Evaporation-induced self-assembly 3.10 400 Surface area; active sites Effect of size and shape of plastics [46] C, N-TiO2 Green bio-inspired synthesis 2.90 428 Surface area; photogenerated carrier separation Effect of pH and temperature in the photocatalytic process [47] Pt-(g-C3N4) Thermal polymerization 2.81 450 Chemical stability; photogenerated carrier separation Hydrogen production of PET [48] Heterojunction construction AgNbO3/Fe2O3 Liquid-phase epitaxy 2.50 580 Photogenerated carrier separation Degradation of PVC [49] g-C3N4/CuFeO2 Solution evaporation / 480−950 Active sites; photogenerated carrier separation Hydrogen production of PET and PLA [50] WO3/g-C3N4 Precipitation method 2.87 400 Surface area; photogenerated carrier separation Degradation of PET [51] VPOM/CNNS Facile electrostatic self-assembly / 460−600 Surface area; photogenerated carrier separation Conversion of PE [52] Electrochemical method KNbO3 Electric field polarization 2.98 410 Photogenerated carrier separation Degradation of PP [53] TiO2 Anodization 3.11 / Surface defects; photogenerated carrier separation; multilayer structure Degradation of PS [54] Cu2O/CuO Anodization 1.84 / Porous structure; photogenerated carrier separation Degradation of PS [30] Cocatalyst loading CN-CNTs-NM In-situ derivatization 2.40 / Porous structure; tight interaction; intensive absorption Hydrogen production of PET [16] Defect engineering Bi4V2O11 Solvothermal method 2.36 / Regulable O-vacancy concentration Conversion of PET [55] Surface photosensitization BiOCl Precipitation method / 218−304 Wide absorption band; tensile strength dropped dramatically Degradation of PS [56] Elementary doping is a commonly used modification method that changes the energy band structures to lower the band gap, enhance visible light absorption, and promote the separation of photogenerated carriers[57]. It can also increase photocatalytic activity through active sites, surface defects, and so on[45,46]. In this field, both metal elements (Pt, Au, Ag, etc.), and non-metal elements (C, N, F, etc.) are commonly selected. For example, F-doped TiO2 lowers the band gap and improves photocatalytic activity by occupying the position of oxygen atoms and displacing them to the interstitial position[26]. To overcome the limitations of single element doping, co-doping has been proposed. Combining doping elements with different properties produces a synergistic effect, resulting in a photocatalytic system that contains the optimal performance of each doping element[47,58].
Heterojunction construction is a common method to promote the separation and transfer of photogenerated carriers, with type-II heterojunctions and Z-scheme heterojunctions being the most commonly employed[59]. Type-II heterojunctions consist of two semiconductors with different energy band structures, achieving band realignment. Under light irradiation, e−/h+ generated on the semiconductors with higher ECB /EVB moves towards the other semiconductors, respectively, thus successfully promoting the separation of electron-hole pairs and enhancing the photocatalytic activity[49]. However, redox reactions occur only on the semiconductors with lower potentials, which limits the redox capacity of catalysts, and like charge repulsion inhibits the transfer of carriers[60]. To overcome this problem, Z-scheme heterojunctions have been proposed. Their energy band structures are similar to those of type-II heterojunctions, but they provide distinct electron transfer paths. Under light irradiation, e- in the low conduction band (CB) position moves to the low valence band (VB) position for recombination, leaving e− with a higher reduction capacity and h+ with a higher oxidation capacity to participate in the photocatalytic reaction[34]. Z-scheme heterojunctions optimize redox potentials, and electron transfer is easier than type-II heterojunctions due to electrostatic attraction[61].
In addition to elementary doping and heterojunction construction, researchers have explored other methods to improve the catalytic performance of photocatalysts, such as the electrochemical method, cocatalyst loading, defect engineering, and surface photosensitization[16,30,53−56]. Liu et al.[53] polarized KNbO3 nanosheet under an external electric field, which significantly enhanced the spontaneous polarization field without changing morphology and structure, thus facilitating the separation of photogenerated carriers. Dominguez-Jaimes et al.[54] used anodization technique to prepare TiO2 photocatalyst with a multilayer structure. The fluorides during the anodization process roughened the catalyst surface and increased the surface defects, promoting the separation of photogenerated carriers. Gong et al.[16] developed an in-situ derived CN-CNTs-NM photocatalyst via NiMo-assisted catalysis route. The II-II conjugated structure between CN and CNTs promoted electron transfer from CN to NiMo, increased the lifetime of photogenerated carriers, and limited the recombination of electron-hole pairs. Liu et al.[55] used Bi4V2O11 photocatalyst with regulable O-vacancy concentration for plastic oxidation and CO2 reduction. Oxygen vacancies (OVs) improved the adsorption and activation of noble gas molecules, and efficiently steered the chemical reaction by lowering the energy barrier. Sarwan et al.[56] added a light-sensitive component, BiOCl, into polystyrene (PS) film to enhance the visible light driving efficiency. The composite film had a wider absorption band than neat PS film, with a maximum absorption band spanning approximately 218−304 nm.
Novel photocatalysts
-
At present, several new photocatalytic materials are being applied in this field, such as metal-organic framework (MOF), and transition metal carbide, nitride, and carbonitride (MXene). MOF is a highly crystalline porous material that offers a high specific surface area, as well as flexible and adjustable structure[62]. The porous structure provides a large surface area to increase the number of active sites involved in the photocatalytic reaction, and interfacial interaction promoted charge separation[27]. MXene is considered a novel two-dimensional photocatalytic material due to its excellent electrical conductivity and high specific surface area. During the reaction process, MXene promotes the separation of photogenerated carriers, acts as robust support, limits the size of photocatalysts, and enhances the adsorption of reactants[24,63].
-
Photocatalytic technology in the treatment and disposal of waste plastics can be categorized into photocatalytic degradation and photocatalytic conversion. The former focuses on breaking down plastics into environmentally friendly small molecules (CO2, H2O, etc.). The latter maximizes the rich hydrocarbon resources in plastics and selectively converts plastics into value-added products[64].
The photocatalytic reaction processes are illustrated in Fig. 4. In brief, when the photocatalyst is irradiated by photons with energy equal to or greater than its band gap energy, e- in the VB is excited and transfers to the CB, leaving corresponding h+ in the VB. Under aerobic conditions, h+ can directly oxidize plastics, or react with adsorbed water molecules to generate hydroxyl radicals (·OH) and H+. In addition to reacting with H+ to produce H2, e− also reacts with oxygen to generate superoxide anion radicals (O2·−), and O2·− further generates other reactive oxygen species (ROS), such as ·OH, H2O2, and singlet oxygen (1O2). Ultimately, these highly oxidizing ROS participate in the photocatalytic reaction, breaking plastics down into small molecules or converting them into value-added products. In comparison, under anaerobic conditions, e− is mainly involved in the reduction of H+. Notably, various ROS are not generated in the absence of oxygen, so plastics are primarily oxidized by h+ and ·OH.
Photocatalytic degradation
-
Currently, studies on the photocatalytic degradation of plastics mainly focus on polyolefins[67,68], PS[69], and PVC[70], with recent studies summarized in Table 2.
Table 2. Recent studies on the photocatalytic degradation of plastics.
Plastics Photocatalysts Light source Time (h) Efficiency Ref. PE BiOCl 250 W Xenon lamp 5 5.38% [66] NiAl2O4 350 W Metal halide lamp 5 12.50% [71] F-TiO2 4.8 W UVA–LED lamp 540 48.00% [23] TiO2/ZnO 365 nm UV light 480 100.00% [67] Bi4Ti3O12 300 W Xenon lamp 6 38.27% [28] PP KNbO3 150 W Xenon lamp / 28.3
mg·gcat−1·h−1
(CO2)[53] ZnO 120 W Tungsten-halogen lamp 336 65.00% [68] PS TiO2 UV lamp 24 99.99% [69] TiO2 UV lamp 50 23.50% [54] CuxO 50 W LED lamp 50 18%~23% [30] CuMgAlTi-R400 50 W Xenon lamp 300 54.20% [20] PVC PVC-TiO2@PANI 30 W UV lamp 720 67.00% [70] Nano-G/TiO2 300 W Medium-pressure UV light 30 17.24% [72] AgNbO3/Fe2O3 350 W Xenon lamp 2 46.53% [49] g-C3N4 Xenon lamp 120 13.08% [73] Polyolefins (e.g., PE and polypropylene (PP)) are extensively used in daily life, accounting for over 50% of global plastic production[74]. The carbon backbone of polyolefins makes them highly stable and difficult to degrade, presenting a significant challenge for photocatalytic degradation. Jiang et al.[66] prepared a hydroxy-rich BiOCl photocatalyst to degrade PE. The surface hydroxyl effectively promoted the generation of ·OH which played an important role in the reaction. After 5 h of light irradiation, the mass loss of PE reached 5.38%, which was 24 times higher than that of BiOCl. Venkataramana et al.[71] prepared NiAl2O4 spinel photocatalysts via co-precipitation (NA), and hydrothermal (NB). The photocatalytic activity of NB exceeded that of NA due to its wider visible light adsorption. NB degraded PE with 12.5% weight loss after 5 h of visible light irradiation. He et al.[67] designed an efficient TiO2/ZnO photocatalyst and added an electron scavenger Na2S2O8 in their experiment on PE degradation. After 480 h, PE was completely degraded, because the electron scavenger significantly increased the generation of ROS. Uheida et al.[68] used glass fiber immobilized ZnO nanorods to degrade PP microplastics suspended in water under visible light, aiming to simulate the real situation of treating plastics in water (Fig. 5). Photocatalysts were fixed in the transparent glass tubes, and water was transported along the tubes, forming a continuous flow system that ensured good contact and interaction between catalysts and plastics. After two weeks, the average volume of PP was reduced by 65%.
Figure 5.
Schematic diagram of the photocatalytic reactor (Source Ref. [68]).
Polystyrene has been produced in large quantities due to its low cost, stable structure, and excellent performance. Its annual production has exceeded 250,000 tons, accounting for about 6% of global polymer production. As one of the least recycled plastics, its photocatalytic degradation has attracted considerable attention. Nabi et al.[69] dispersed PS nanospheres on TiO2 nanoparticle films prepared with Triton X-100 (TXT) and conducted solid-phase photocatalytic degradation. After 24 h of UV irradiation at 245 nm, 5 mm PS nanospheres achieved nearly complete degradation (99.99%), which was significantly higher than the degradation achieved in the liquid phase. This improvement was attributed to the solid-solid interface that promoted the separation of photogenerated carriers and allowed ROS to attack plastics without barriers. In addition, the solid-phase reaction system avoided the release of toxic by-products into the solution, and catalysts were easily recycled at the end of the reaction[73]. Acuña-Bedoya et al.[30] prepared an immobilized CuO/Cu2O photocatalyst using the anodization technique for the degradation of PS. During the anodization process, fluoride ions in the solution were adsorbed on copper to prevent the direct oxidation of Cu (0) to Cu (II), resulting in the formation of a catalyst with a mixed and porous structure. After 5 h of visible light irradiation, the elimination of PS reached 23.5%.
Polyvinyl chloride is a typical thermoplastic polymer widely used in industries such as construction, medicine, and automobile. In real life, PVC is difficult to degrade due to its high content of chlorine and harmful additives. Therefore, researchers have attempted to incorporate photocatalysts into plastics to promote closer contact, thereby increasing the reaction rate and enabling the spontaneous degradation of PVC-based plastics under environmental conditions[73,75]. However, it is worth noting that this method is still in the laboratory stage, and no commercial products are currently available[23]. Zhang et al.[72] added nano-graphite (Nano-G) and TiO2 into PVC to prepare Nano-G/TiO2/PVC composite film. Nano-G with a special structure allowed for the uniform distribution of TiO2 in the polymer, and its excellent conductivity facilitated the separation and transfer of photogenerated carriers, thereby enhancing the photocatalytic activity of TiO2. After 30 h of light irradiation, the weight loss of this film reached 17.24%, which was higher than that of Nano-G/PVC film and TiO2/PVC film. However, the addition of catalysts decreased the tensile strength and elongation at the break of the composite film, possibly because catalysts hindered the connection between the polymer monomers. Gou et al.[73] used urea as a precursor to prepare g-C3N4 by thermal polymerization method, and added them into PVC to obtain PVC/g-C3N4 composite film. After 120 h of light irradiation, this film exhibited the highest weight loss of 13.08%, which was 58% higher than that of pure PVC film. Moreover, the mechanical property of PVC was enhanced after g-C3N4 incorporation. The tensile strength increased by 45% and the elongation at break increased by 72%.
Based on the experimental studies, the mechanism of photocatalytic degradation of PE, PP, PS, and PVC can be summarized in Fig. 6. Firstly, ·OH preferentially attacks the C-H bond in the polymer chain, causing bond cleavage and the formation of a carbon-centered radical. This radical then reacts with O2 to form a peroxy radical. The peroxy radical subsequently breaks the C-H bond on another polymer chain, abstracting one H atom and forming hydroperoxide species. The O-O bond in the unstable hydroperoxide species then breaks, producing a free oxy radical and ·OH. Finally, the free oxy radical continues to be oxidized and disintegrated into various oxygen-containing intermediates (e.g., ketone, aldehyde, and carboxylic acid), eventually being degraded into CO2 and H2O.
Photocatalytic conversion
-
The focus of research on photocatalytic conversion has primarily been on polar polyester plastics, such as PET and polylactic acid (PLA). PET, the most widely used polyester, can be found in products like textiles, packaging bags, and beverage bottles[67]. Currently, its global annual production has exceeded 50 million tons. PLA, a leading biodegradable plastic, is commonly used in the production of films, medical devices, and textiles. Its market share reached USD
2.1 billion in 2021 and is expected to grow to USD$ 4.1 billion by 2026[28]. In an alkaline solution, PET can be easily hydrolyzed into monomers, primarily terephthalic acid (TPA), and ethylene glycol (EG). However, TPA is difficult to photooxidize, so only EG participates in the photocatalytic reaction to generate value-added products[45]. The hydrolysate of PLA is lactic acid[77]. For example,$
Du et al.[35] conducted photocatalytic experiments on PET and PLA using MoS2-tipped CdS nanorods (MoS2/CdS). To improve the conversion rate, 1.5 g PET or PLA was added to 60 mL of 10 M KOH and stirred at 40 °C for 48 h. After centrifugation, 60 mL supernatant was used for subsequent experiments. After 5 h of light irradiation, EG hydrolyzed from PET was oxidized to carboxylates, with cumulative concentrations of formate and acetate reaching 5.96 ± 0.02 and 0.95 ± 0.01 mmol·L−1, respectively. Lactic acid hydrolyzed from PLA was oxidized to formate, with an accumulated concentration of 5.37 ± 0.67 mmol·L−1.In addition to hydrolyzing PLA to lactic acid in an alkaline solution, researchers have proposed a reaction pathway to convert PLA into chemicals in an aqueous solution[78]. At the beginning of the reaction, ROS are likely to preferentially attack the C-O bond on the polymer chain due to its relatively low bond energy (326 kJ·mol−1). As the polymer continues to be oxidized, other bonds are broken, leading to the formation of oxygen-containing intermediates that eventually yield chemicals. Qin et al.[79] prepared ZnO/UiO66-NH2 heterojunction photocatalyst to convert PLA into acetic acid, achieving a yield of 14.4% and a selectivity of 91.6%. The conversion rate of PLA was 57.10 mg·gcat−1·h−1.
Unlike PET and PLA, PS, PVC, and polyolefins are difficult to hydrolyze, so they directly participate in the photocatalytic reaction. For PS, researchers usually use hydrogen atom transfer (HAT) photocatalysts to treat the inert C-H bonds of plastics in organic solvents. During the reaction, HAT photocatalyst abstracts hydrogen atom on the polymer chain to break the C-H bond, and then reacts with O2 to realize catalyst regeneration. The plastic radical formed in the process reacts with O2 to generate peroxy radical. Upon further irradiation, the C-C bonds are broken, producing various intermediates that are finally converted into chemicals. Li et al.[80] chose fluorenone as an HAT photocatalyst, and successfully deconstructed PS into benzoic acid with yields of 30% at 16 h and 38% at 48 h under mildly acidic conditions (Fig. 7). Small amounts of other aromatic products were also obtained, including ethyl benzoate (10%), acetophenone (6%), and benzaldehyde (1%), with the remaining 40% being aromatic functionalized oligomers. On this basis, Nikitas et al.[81] chose anthraquinone as an HAT photocatalyst, achieving 28.2% yield of benzoic acid from PS under 390 nm LED irradiation for 48 h under open-air conditions. This system didn't require an acidic environment, and benzoic acid was isolated through simple base-acid wash and extraction. Moreover, this catalyst showed excellent photocatalytic performance for the degradation of commercial plastics, yielding 51.4% for a PS-based plastic spoon and 58.8% for a big transparent plastic cup.
Figure 7.
Schematic illustration of the photocatalytic deconstruction of PS, and the yields of various products over the reaction time ( Source Ref. [80]).
For PVC, its photocatalytic conversion begins with ROS attacking the C-Cl bond on the polymer chain to generate carbon-centered radical. The C-C bonds of unstable carbon-centered radical are further broken, leading to the formation of a series of oxygen-containing intermediates that are eventually converted into chemicals[79,82]. Qin et al.[82] used CDs/Zr-MOF photocatalysts fabricated by carbon nanodots (CDs) and MOF to convert PVC into acetic acid successfully (Fig. 8). When the mass fraction of CDs was 10%, the PVC conversion reached approximately 76.5%. However, a further increase in the mass fraction of CDs resulted in a decrease in PVC conversion. This reduction may be attributed to an excessive number of CDs, which could affect light absorption and promote the recombination of photogenerated carriers. In addition, the products were found to be a complex mixture of plastic fragments and small molecular weight species, complicating the analysis of their selectivity.
Figure 8.
Elementary pathways for the photocatalytic conversion of PVC to acetic acid, and PVC conversion activity over CDs/Zr-MOF (Source Ref. [82]).
For polyolefins, researchers have proposed a two-step process to achieve their photocatalytic conversion. Step 1: Under light irradiation, plastics are oxidized to CO2. Step 2: CO2 is reduced to chemicals via ·COOH intermediates. Jiao et al.[29] successfully converted PE and PP into C2 fuels in the simulated natural environment using Nb2O5 photocatalyst. Firstly, PE and PP were completely degraded into CO2 within 40 h and 60 h, respectively. Then, CO2 was reduced to CH3COOH through photoinduced C-C coupling of ·COOH intermediates, with average production rates of 47.4 and 40.6 mg·g−1·h−1, respectively. The amount of CH3COOH and its generation rate were comparable to those obtained from the photoreduction of pure CO2 in water, and FTIR spectra also confirmed that CH3COOH was not derived from the direct photoconversion of plastics. Under ambient conditions, Xu et al.[83] used Co-Ga2O3 nanosheet photocatalyst to convert PE bags into syngas (CO and H2), an important feedstock for producing chemicals and fuels. In the photocatalytic reaction, H2O was reduced to H2, and plastics were oxidized to CO2 which was further reduced to CO. The production rates of H2, CO2, and CO were 647.8, 419.3, and 158.3 μmol·g−1·h−1, respectively, which were 1.6, 1.6, and 1.9 times higher than those of pure Ga2O3. The reaction rate was primarily determined by the reduction rate of CO2.
Selectivity is one of the important metrics for photocatalytic conversion (Table 3). During the photocatalytic reaction, plastics are oxidized by h+ or various ROS. Multiple oxidation pathways can lead to side reactions that reduce the selectivity of target products. Therefore, researchers have designed advanced photocatalysts to increase the generation of certain ROS, achieving specific reaction pathways and eventually improving the selectivity of products. Wu et al.[78] proposed a solution plasma strategy to prepare a Pt-loaded Bi12O17Cl2 photocatalyst with a large number of OVs that could adjust the energy band structure. This catalyst had a narrow band gap and a negative ECB to promote charge transfer. Besides, its strong reduction capacity facilitated the generation of ·O2·−, successfully reducing the oxidation pathways. Finally, the yield rate and selectivity of acetic acid generated from PVC were 6.07 mg·gcat−1·h−1 and 94%, respectively. Han et al.[84] prepared carbonized polymer dots-graphitic carbon nitride (CPDs-CN) to conduct photocatalytic experiments on PET. After 8 d of light irradiation, the yields and intermediate selectivity of glycolic acid and acetic acid were 383 μmol, 27%, and 554 μmol, 40%, respectively. Intermediate selectivity was defined as the ratio between the mole of one specific product and the total products. Using sodium terephthalate as a probe, it was found that ·OH was not present and h+ played a major role in the oxidation reaction, which achieved the selection of products.
Table 3. Recent studies on the selectivity of photocatalytic conversion.
Plastics Photocatalysts Light source Products Activity Selectivity Ref. PS F-g-C3N4 250 W Hydrargyrum lamp Acetophenone 72.80% conversion 81.60% [85] Benzoic acid 18.40% g-C3N4 300 W Xenon lamp Aromatic oxygenates 100.00% conversion 59.00% [41] PVC CDs/Zr-MOF 300 W Xenon lamp Acetic acid 2.56 mg·g−1·h−1 ~76.50% [82] Pt-decorated Bi12O17Cl2 300 W Xenon lamp Acetic acid 6.07 mg·g−1·h−1 94.00% [78] PLA 300 W Xenon lamp Formic acid 47.43 mg·g−1·h−1 55.10% ZnO/UiO66-NH2 300 W Xenon lamp Acetic acid 14.40% yield 91.60% [79] Ni2P/ZnIn2S4 white LED light source Pyruvic acid 745.90 μmol·g−1·h−1 >90.00% [86] PAN@(FeCoNiCuZn)WO4 300 W Xenon lamp Acetic acid 38.51 mg·g−1·h−1 73.00% [87] PET CPDs-CN 300 W Xenon lamp Glycolic acid 383.00 μmol/8 d ~27.00% [84] Acetic acid 554.00 μmol/8 d ~40.00% In addition, improving external environmental conditions can also enhance the selectivity of products[19]. Cao et al.[41] used g-C3N4 photocatalyst to convert PS into aromatic compounds under visible light irradiation (Fig. 9). Considering the self-oxidation competition, reaction temperature, O2 pressure, and metal additives, researchers optimized the reaction system, achieving product selectivity of up to 59% and a conversion rate close to 100% after 24 h. To prevent overoxidation, they set up a circulatory system that retained PS and catalysts, drained the solution through a filter, and replenished an equal volume of solvents. Ultimately, 90% of plastics were converted into aromatic oxygenates (74% benzoic acid, 15% acetophenone, and 11% benzaldehyde). Researchers also found that the weight hourly space velocity (WHSV) influenced the selectivity, with a higher selectivity for benzaldehyde (51%) and acetophenone (31%) under high WHSV, and for benzoic acid (74%) under low WHSV.
Figure 9.
Schematic diagram of PS oxidation reaction, and its activity and selectivity at different WHSV (Source Ref. [41]).
In summary, the reaction pathways for the photocatalytic conversion of different types of plastics are as follows (Fig. 10): For plastics that are easily hydrolyzed (e.g., PET and PLA), their hydrolysis products typically participate in the oxidation reaction to produce chemicals[86]. PLA can also directly start the oxidation reaction through chain scission[79]. For PS and PVC that are difficult to hydrolyze, the bonds with the lowest bond energy in the polymer chains are attacked preferentially, cleaving the bonds to form plastic radicals[81]. Plastic radicals are further oxidized into plastic fragments or lower molecular weight oxygen-containing intermediates (primarily with hydroxyl and carbonyl), and ultimately chemicals are obtained. Non-polar plastics, like polyolefins, have stable C-C bonds and are not easily activated. Therefore, most of them are first photodegraded into CO2, and then CO2 is photo-reduced into chemicals[83]. Besides, the dibasic acids generated from PE pretreated by acid are successfully photooxidized[35].
Hydrogen production
-
Hydrogen is considered a promising clean energy source due to the ongoing energy shortage and severe environmental pollution. In 1972, Fujishima & Honda[88] first proposed the Honda-Fujishima effect, which involves the photolysis of water on the TiO2 surface under UV irradiation. This groundbreaking discovery spurred extensive research into photocatalytic water splitting and H2 production[89]. However, the slow kinetics of photocatalytic water oxidation limits the efficiency of H2 production. To accelerate the oxidative half-reaction, sacrificial agents are often used, but this increases the overall cost[90]. Recent studies have revealed that cheap and abundant plastics can serve as sacrificial agents in the photocatalytic reaction, enabling both H2 production and waste plastic disposal[91−93]. PLA and PET are the main raw materials used in these studies[77,94] (Table 4).
Table 4. Recent studies on the hydrogen production.
Photocatalysts Plastics Light source Amount of photocatalysts H2 production
(mmol·g−1·h−1)Year Ref. CdS/CdOx 50 mg·mL−1 PLA in 10 M KOH Simulated solar light 1.0 nmol 64.300 2018 [36] CNx|Ni2P 25 mg·mL−1 PLA in 1 M KOH Simulated solar light 3.2 mg 0.178 2019 [40] MoS2/CdxZn1-xS 25 mg·mL−1 PET in 10 M NaOH 300 W Xenon lamp 10.0 mg 15.900 2020 [21] MXene/ZnxCd1-xS 50 mL PET 300 W Xenon lamp 10.0 mg 14.170 2021 [24] Pt-loaded BSx−CNs 10 mg·mL−1 PLA in 1 M KOH 300 W Xenon lamp 10.0 mg 1.890 2021 [94] CN-CNTs-NiMo 50 mg·mL−1 PET in 5 M KOH 500 W Xenon lamp 10.0 mg 0.086 2022 [16] Ag2O/Fe-MOF 5 mg·mL−1 PET in DI water 300 W Xenon lamp 100.0 mg 1.900 2022 [27] Au/TiO2 50 mg·mL−1 PET in 3 M KOH 160 W high-pressure
UV mercury vapor lamp500.0 mg 0.001 2023 [45] d-NiPS3/CdS 10 mg·mL−1 PLA in 2 M KOH 300 W Xenon lamp 1.0 mg 39.760 2023 [37] ZnIn2S4 25 mg·mL−1 PET in 1 M KOH CEAULight 7.5 mg 143.600 2023 [77] CdS/NiS 100 mg·mL−1 PLA in 10 M KOH 300 W Xenon lamp 5.0 mg 62.900 2023 [22] Pt-loaded g-C3N4 20 mg·mL−1 PET in 5 M NaOH 100 W Xenon lamp 100.0 mg 7.330 2023 [48] Uekert et al.[36] used cost-effective CdS/CdOx quantum dots to produce H2 from PLA and PET under visible light irradiation. Through optimization of experimental conditions, the maximum H2 production rates were 64.3 ± 14.7 and 3.42 ± 0.87 mmol H2 g−1·h−1, respectively. The total amount of H2 production beyond 22 h was 3.09 ± 0.15 and 0.21 ± 0.04 mmol H2 g−1. On this basis, Uekert et al.[40] conducted an experiment using a nontoxic CNx|Ni2P photocatalyst. After 50 h of light irradiation, H2 production from PLA and PET reached 178 ± 12 and 82.5 ± 7.3 μmol H2 g−1, respectively. The researchers also conducted experiments with polyester microfibers and a PET bottle. When other factors stayed identical, H2 production rates were 2.67 ± 0.25 and 2.87 ± 0.16 μmol H2 g−1·h−1, respectively. The reduced photocatalytic efficiency of real-life plastics might be attributed to the presence of additives that hindered the hydrolysis of plastics made the photocatalytic reaction more challenging.
Aiming to address the practical application challenges of photocatalytic H2 production (catalyst lifetime, recyclability, competitive absorption, etc.), Uekert et al.[95] developed a scalable CNx|Ni2P panel by a facile drop-casting and low-temperature method, which prevented the deposition of catalysts, facilitated their recycling, and reduced the competition with turbid solution components for light absorption. Under back irradiation, the panel achieved a maximum H2 production rate of 156 ± 15 μmol H2 m−2·h−1, comparable to slurries with the same CNx|Ni2P loading. When the panel was expanded from 1 to 25 cm2, the efficiency of H2 production dropped by three-fold, likely due to mass transport and irregularities in the larger panel. The stability of the 1.92 mg·cm−2 CNx|Ni2P panel was also tested, and it retained over 70% activity after four cycles in 0.5 M aq. KOH, indicating that the panel was highly stable. Linley et al.[96] deposited CNx|Pt on a hollow glass microsphere (HGM), enabling it to float in the aqueous phase. This method enhanced light absorption in the turbid solution and facilitated catalyst recycling. In a small reactor of 4.9 cm2, the mass recovery of HGM/CNx|Pt was 61.4% ± 3.7% after 10 trials, and the separation was rapid with a clear solution obtained over 10 min. After expanding the reactor area to 217 cm2, the activity of HGM/CNx|Pt decreased slightly to 93.4% ± 11.4% of the initial activity, indicating that this system could be scaled up without significant loss of areal activity.
-
The material flow in the photocatalytic disposal of plastics involves the input of plastics, the role of catalysts, and the generation of products. Plastics are usually input into the system in a solid-state to react with h+/ROS, leading to the production of small molecules or value-added products. Incomplete disposal of solid plastics and deactivated catalysts require further collection and treatment. In terms of energy flow, light energy serves as the primary energy source driving the photocatalytic reaction, and light intensity directly influences the reaction rate. Light energy is converted into chemical energy by exciting the generation of e− and h+, which in turn produces a series of products. Additionally, part of the energy is converted into heat.
It can be seen that the photocatalytic reaction process is complex, with several influencing factors that can be classified into plastic-type, catalyst structure, pretreatment method, and reaction environment. Among them, plastic-type is the most important factor, as it decides which catalyst structure, pretreatment method, and reaction environment to choose, as well as the type of products. Polyolefins, PS, and PVC contain inert C-C and C-H bonds, making them highly stable and difficult to activate, degrade, and convert[97]. Their hydrophobicity also limits their dispersion in solution, reducing the contact with photocatalysts. In comparison, polyesters (e.g., PET and PLA), as polar plastics, are more easily hydrolyzed into the corresponding monomers that can subsequently participate in the photocatalytic reaction. These plastics are also preferred feedstocks for photocatalytic hydrogen production. In addition, the structure and morphology of plastics significantly impact the photocatalytic process. Studies have shown that compared to film-shaped plastics, small-size plastics are easier to degrade, which is related to low illumination and oxygenated reaction medium[46]. Compared with light-colored plastics, dark plastics have stronger light absorption ability, which enhances the surface catalysis of plastics[66].
The second influencing factor is catalyst structure, mainly including energy band structure, morphological structure, and doping percentage. These aspects can be controlled by the synthesis route, thereby impacting the photocatalytic performance of catalysts[45]. Regarding the energy band structure, suitable redox potentials are essential for the catalysts to drive the reaction. For instance, photocatalytic conversion of plastics and hydrogen production requires that the CB potential of catalysts is more negative than the reduction potential of H2, and the VB potential is more positive than the oxidation potential of plastics[98]. Therefore, catalysts with strong oxidation capacities are ideal for plastics containing stable C-C bonds. For short-chain plastics or small molecules obtained after pretreatment, catalysts with slightly weaker oxidation capacities may be sufficient. In addition, the band gap size of catalysts affects their light absorption range. To increase the utilization of visible light, narrow-band catalysts are preferable over wide-band catalysts. The internal electric field of catalysts also influences the migration rate of photogenerated carriers. By enhancing the internal electric field, the separation of electron-hole pairs can be significantly improved[99]. Regarding the morphological structure, taking TiO2 as an example, TiO2 nanoparticle film made with TXT has a special film texture and surface hydrophilicity, so its photocatalytic activity is higher than films made with water or ethanol[69]. TiO2 with multilayer structure has higher photocatalytic activity than barrier structure or nanotubular structure, because it improves the contact with plastics by increasing the development surface, and its surface defects facilitate the separation and transfer of photogenerated carriers[54]. Experiments with various doping percentages have shown that the increase of additive amounts can enhance charge separation efficiency and inhibit the recombination of electron-hole pairs. However, excessive doping may lead to problems such as occupying the active sites and affecting the light absorption, ultimately resulting in a decrease in photocatalytic performance[49].
The pretreatment method plays an important role in improving photocatalytic efficiency, mainly including alkaline/acidic pretreatment, hydrothermal pretreatment, and plasma pretreatment[35,36,65,97]. At present, the most commonly used method is alkaline pretreatment, especially for polar plastics like polyesters. For example, PET can be hydrolyzed to TPA and EG in an alkaline solution. TPA belongs to aromatic compounds that are difficult to photooxidize, but it can be precipitated by adding acid to realize separation[36]. Purified TPA can then be used to produce MOFs with higher surface areas and enhanced water adsorption performance via the solvothermal method[100]. PLA is easily hydrolyzed to lactic acid in an alkaline solution. Non-polar plastics like polyolefins, are unsuitable for alkaline pretreatment. In these cases, acidic pretreatment has been employed on PE to obtain a mixture of short-chain carboxylic acid[35]. However, both alkaline pretreatment and acidic pretreatment have the same disadvantages, including high cost, difficulty in recycling, and possible negative impacts on the environment. Researchers have pretreated PET with hydrothermal pretreatment, which also significantly increased the weight loss of plastics[65]. For non-polar plastics, plasma pretreatment can be used to graft oxygenated groups onto their surfaces, making them become polar polymers with hydrophilicity, which enhances the interaction with catalysts[97]. Notably, the pretreatment method may also influence H2 production. For instance, higher pretreatment temperature and KOH concentration are beneficial to produce H2 in the photocatalytic reaction of PET[45].
In addition, the photocatalytic efficiency is influenced by the reaction environment, mainly including light intensity[101], O2[102], solvents, temperature, and pH. Different reaction conditions can affect the type and function of active species in the photocatalytic process, resulting in various reaction pathways and photocatalytic efficiency[102]. Regarding the reaction atmosphere, under aerobic conditions, a range of highly oxidizing ROS are generated during the photocatalytic reaction. However, they are non-selective and unable to produce specific chemicals. In contrast, only ·OH and h+ are generated to oxidize plastics under anaerobic conditions. While this system exhibits reduced oxidation capacity, it offers a better selectivity of products and can enhance H2 production. For reaction solvents, the efficiency in an aqueous solution is generally lower than that in an alkaline/acidic solution. Researchers have used organic solvents in the photocatalytic reaction of PS[80]. When plastics are dissolved in organic solvents, their contact with catalysts is enhanced, and organic solvents can influence both the activity and selectivity of the system. In terms of temperature and pH, taking PE as an example, low temperature, and low pH have positive effects on its degradation[47]. Low temperatures can enhance the interaction between catalysts and plastics by increasing the surface area through fragmentation. Low pH introduces H+ into the system, which facilitates the generation of ROS to further degrade plastics. In addition, there may be a synergistic effect between reaction environments. Compared with temperature or pH, their interaction may highly enhance the photocatalytic efficiency of plastics[43].
-
Plastics are omnipresent in our daily lives, and their continuous production, usage, and improper disposal have made waste plastics a major global environmental issue, posing significant threats to both human and animal life. Therefore, there is an urgent need for effective treatment and disposal technologies. Photocatalytic technology has emerged as a promising technology due to its direct utilization of solar energy, mild reaction conditions, and environmental friendliness. Photocatalytic technology offers environmental advantages for small-scale plastic disposal, particularly in treating laboratory waste plastics and localized plastic pollution. However, its large-scale application faces challenges related to efficiency and cost, making it less competitive in plastic recycling and the circular economy. As a result, it is currently used as a supplementary technology, supporting methods like mechanical recycling. With catalyst development and process optimization, photocatalytic technology holds significant potential for broader application in targeted fields. Its future is rich with opportunities, yet accompanied by notable challenges.
Mechanistic study plays an important role in the whole research process. Currently, most studies focus on polyesters as research materials, primarily because the functional groups make their backbone easy to break, which facilitates subsequent redox reactions. In comparison, fewer experiments have been conducted on plastics containing stable C-C bonds. A deeper understanding of the mechanism can help researchers design more efficient photocatalysts and reaction systems, thereby enhancing the activity of reaction and the selectivity of products, and addressing the puzzle of limited plastics. In addition, appropriate characterization methods are essential for more accurately studying the behavior of reactants during the photocatalytic process, so it is necessary to explore advanced identification and quantitative methods.
Laboratory researches often focus on pure plastics. However, real-life plastics contain various chemical additives (e.g., plasticizers, flame retardants, and antioxidants), whose effects on photocatalytic activity, selectivity, and product quality remain unclear. In addition, more attention should be given to plastic waste mixtures with external impurities, because efficient treatment and disposal of these mixtures can reduce sorting and disposal costs.
Effective photocatalysts should have energy band structures that can absorb visible light, facilitate redox reactions, and effectively improve the separation of electron-hole pairs. However, the photocatalysts currently used in this field are relatively limited and may only be suitable for specific types of plastics. Therefore, there is a need to explore photocatalysts with enhanced performance, higher stability, and broader applicability for the disposal of real-life plastics. What's more, simplifying the preparation and recycling processes of catalysts is essential to achieve large-scale production and practical application.
Traditional plastics are difficult to degrade and recycle, resulting in the waste of valuable hydrocarbon resources. Thus, it is necessary to design new degradable plastics that can break down more easily under mild conditions, while still maintaining their mechanical properties. For example, reducing the reliance on fossil fuels or introducing special components into plastics may help induce and accelerate the photocatalytic reaction.
The potential risk of secondary pollution should be carefully considered. During the photocatalytic degradation and conversion, small molecules such as aldehydes, ketones, and organic acids are produced, some of which may be toxic. Particularly for complex polymers (e.g., PVC and polyesters), toxic gases or hazardous substances (e.g., chlorides and benzene compounds) may be released. If these products are not treated in time, they could cause harm to the environment and human health. Besides, solid waste plastics and catalysts after reaction may become secondary pollutant sources if not disposed of properly. Therefore, it is necessary to optimize the photocatalytic processes, improve the selection and stability of catalysts, strengthen control over reaction conditions, and take effective monitoring and treatment measures for emission.
The circular economy is an important pathway to address plastic pollution. It emphasizes the entire lifecycle of plastics, from design to end-of-life recovery, aiming to minimize the generation of waste plastics and their environmental impacts. Governments have proposed several strong policy measures, such as promoting the transition to a circular plastic economy and moving away from single-use plastics. China has proposed to achieve carbon peaking by 2030 and carbon neutrality by 2060, so the treatment and disposal of waste plastics become an important guarantee for realizing these goals. It is necessary to further advance the circular economy and support the achievement of dual-carbon goals through the recycling, processing, and utilization of waste plastics.
This work was supported by the National Natural Science Foundation of China (52176197; 52322005; 52100156; 52400179), Young Scientific and Technological Talents (Level One) in Tianjin (QN20230114), Tianjin Science and Technology Committee (24JCJQJC00140), Postdoctoral Fellowship Program of CPSF (GZC20241199), and China Postdoctoral Science Foundation (GZC20241199; 2024M762352).
-
The authors confirm contribution to the paper as follows: conceptualization: Cheng Z, Wang J, Li N; supervision: Wang J, Zhang Y, Li N, Yan B, Chen G; writing - original draft: Guan B; writing - review & editing: Cheng Z, Wang J, Zhang Y, Li N, Sun Y, Yan B, Chen G. All authors reviewed the results and approved the final version of the manuscript.
-
Data sharing not applicable to this article as no datasets were generated or analyzed during the current study.
-
The authors declare that they have no conflict of interest.
- Copyright: © 2025 by the author(s). Published by Maximum Academic Press, Fayetteville, GA. This article is an open access article distributed under Creative Commons Attribution License (CC BY 4.0), visit https://creativecommons.org/licenses/by/4.0/.
-
About this article
Cite this article
Cheng Z, Guan B, Wang J, Zhang Y, Li N, et al. 2025. Application of photocatalytic technology in the treatment and disposal of waste plastics: a review. Progress in Reaction Kinetics and Mechanism 50: e002 doi: 10.48130/prkm-0025-0002
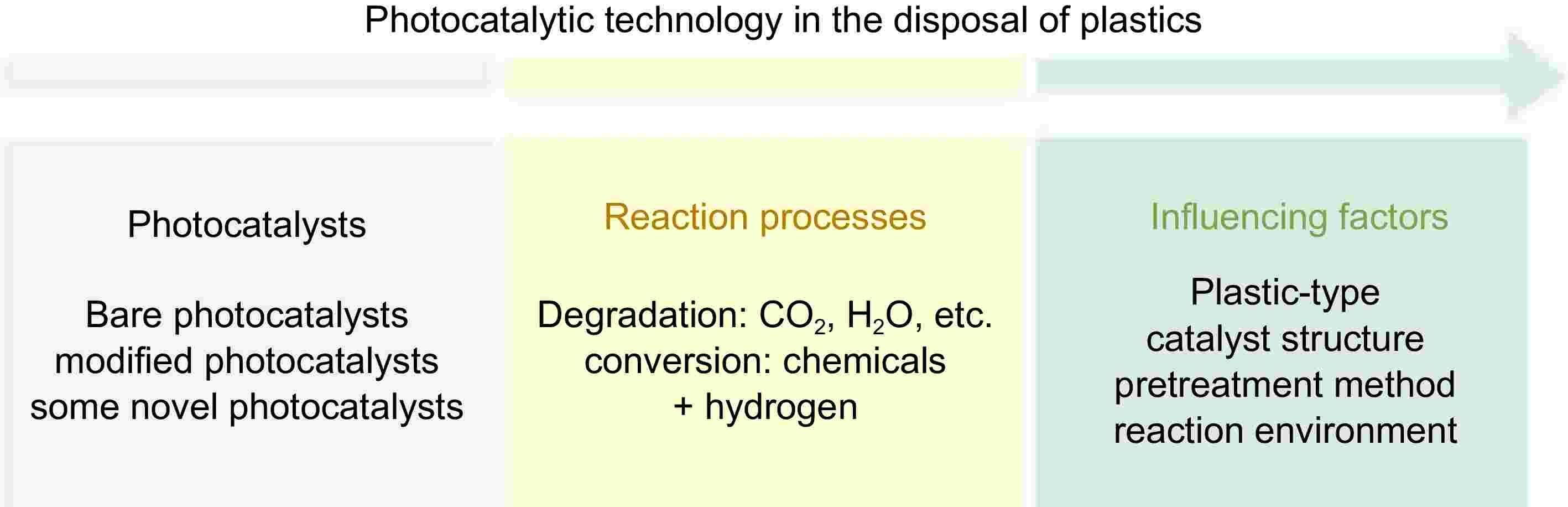