-
Crimean-Congo Hemorrhagic Fever (CCHF) is a tick-borne disease prevalent in Afghanistan, with a Case Fatality Ratio (CFR) of 10% to 50%. Its incidence is rising in northeast Afghanistan, with animals as the primary source of infection. First identified in the 1940s in the Crimean Peninsula, it is caused by an enveloped negative-sense single-stranded RNA virus belonging to the Bunyaviridae family, Nairovirus genus. The main mode of transmission is through ticks, especially the Hyalomma species[1].
Wild animals, such as rabbits, hedgehogs, and certain rat species, serve as reservoirs for CCHF in different regions. Domestic animals like cattle, sheep, goats, camels, horses, dogs, donkeys, and poultry also act as reservoirs and amplifying hosts[1]. They can be asymptomatically infected or harbor infected ticks, with cattle being particularly important for CCHFV transmission[2]. Detecting CCHF viral RNA in clinical samples is crucial during the acute phase, especially before symptoms appear when antibody detection isn't feasible. Rapid and reliable diagnostic methods are essential due to high fatality rates, pathogenicity, and potential human-to-human transmission[3,4]. Early, accurate detection and monitoring of viral load are crucial for managing cases and ensuring biosafety, given the absence of specific treatment or approved vaccines[5]. CCHF is a major public health concern in Eastern Europe, Africa, the Middle East, and Asia, where the Hyalomma tick is common. People involved in animal husbandry and slaughtering, especially in rural areas of Afghanistan, face significant risk[6]. Transmission of CCHFV happens through tick bites, contact with crushed infected ticks, animal secretions or blood on injured skin or mucosa, and exposure to contaminated surgical instruments[1,7].
In Afghanistan, CCHF is mainly reported among livestock workers, but cases have also been documented among healthcare personnel, veterinarians, meat inspectors, butchers, livestock traders, hunters, farmers, ranchers, and the general population[1]. Occupational exposure to infected animals and humans increases the risk of contracting CCHF. The first case was reported in 1998 in Takhar province, northeast Afghanistan. The WHO noted a substantial rise in cases, with 30 reported in 2018 and 947 from all 34 provinces in 2023, leading to 100 fatalities. Afghanistan is an endemic area for CCHF, facilitated by the Hyalomma tick's ecological range.
CCHF prevalence rises throughout the year, particularly during Eid-Al-Adha, a religious holiday characterized by widespread animal sacrifices and unprofessional slaughtering in rural/urban areas[5]. Eid-ul-Adha is an annual religious festival during which millions of farm animals, including goats, cows, sheep, and camels, are slaughtered. This period, typically falling between June and September, is considered the most susceptible time for disease contraction, particularly Crimean-Congo Hemorrhagic Fever (CCHF). The preference for self-slaughter due to the unavailability of butchers and the convenience of house slaughtering by professional butchers contributes to animal-to-human disease transmission. Notably, CCHF is primarily confined to rural areas of Afghanistan[8,9].
In 2022, Afghanistan was among the countries with the highest number of CCHF cases reported by the WHO. The number of confirmed cases has been on the rise in Afghanistan recently, but the capacity for laboratory testing and case management remains limited[5,10]. Various lab tests diagnose CCHFV, such as ELISA, serum neutralization, antigen detection, virus isolation, and RT-PCR. RT-PCR is preferred for its simplicity, specificity, and sensitivity[4].
Therefore, this study aimed to investigate the CCHF virus in Afghanistan, focusing on identifying its primary reservoirs and transmission factors. We conducted molecular and seroprevalence analyses, examined tick morphology, and reviewed national surveillance data from 2007 to 2024. We assessed seroprevalence and molecular detection in blood and tick samples from domestic animals in Kunduz and Takhar provinces. Our findings could guide future surveillance efforts to address this public health threat.
-
Kunduz province, strategically located at a border intersection with Takhar, Baghlan, Balkh, and Tajikistan, is a pivotal crossing point. Kunduz has a population of 1,308,389 residents, comprising both rural and urban dwellers[11].
Takhar, situated in the Northeastern Region of Afghanistan, is one of 34 provinces. The province has a population of 1,109,573 inhabitants, including rural and urban populations. The main occupations in these provinces encompass agriculture, animal husbandry, clothing production, labor, carpet weaving, and business[11].
Study design
-
A cross-sectional study was conducted from January to March 2024 in the Kunduz and Takhar provinces, Afghanistan. With an expected prevalence of 50%, a sample size of 427 livestock per province was calculated at a 95% confidence level and 5% precision.
Districts, farms, and villages were chosen based on WHO-identified high outbreak areas. Animal species (cows, sheep, camels, goats, and chickens) were randomly selected, regardless of age, sex, or breed, without tagging animals on farms. A systematic sampling method ensured each animal had an equal chance of selection, with owners consenting before sampling.
Blood sample collection and processing
-
Four hundred and eighty blood samples were collected equally from four districts each in Kunduz and Takhar provinces. Trained veterinarians assisted in drawing 5 ml blood samples from cattle, sheep, camels, goats, and chickens via the jugular vein using BD Vacutainer 10 ml Hematology (K₃EDTA) tubes, regardless of age. Samples were promptly transported on dry ice to the Central Veterinary Diagnostic and Research Laboratory (CVDRL) to maintain cold chain integrity. Upon arrival, serum was obtained through centrifugation, transferred to labeled 5 ml cryogenic vials, and stored at −20°C until further serological and molecular testing.
Detection of CCHFV RNA in serum samples and amplification
-
CCHF-suspected samples were meticulously investigated for CCHF RNA presence. Total RNA extraction from serum samples utilized the Viral Nucleic Acid Isolation Kit from BioPerfectus Technologies. Extracted RNA was reverse transcribed to cDNA, and amplification was conducted using the one-step RealStar® 1.0 RT-PCR kit from Altona Diagnostics (Germany) on an AriaMx real-time PCR machine.
Tick sample collection and processing
-
Ticks were systematically collected from livestock farms, including cattle, sheep, camels, goats, and chickens, with tick collectors wearing full-body protective clothing. Animals underwent thorough examinations to locate ticks in specific areas. Ticks were carefully removed using blunt forceps and transferred into labeled safety-lock Eppendorf tubes®. Live ticks were transported to the CVDRL in Kabul for morphological examinations, then stored at −80 °C for mRNA extraction and further analysis.
Tick identification
-
Ticks were identified based on their geomorphological features under a light stereomicroscope using a multiple electronic entomology key[12]. The ticks were identified up to the species level based on morphological characteristics of the ticks for species identification and recorded respectively.
Detection of antibodies directed against CCHFV and RNA extraction
-
The sera were serologically tested as described by Schuster et al.[13]. All samples were first tested in an adapted commercial species-specific indirect CCHFV-IgG ELISA. In the adapted commercial species-specific indirect CCHFV-IgG ELISA, the samples with an OD value > 0.7 were considered positive. In a second step, samples with divergent results were run in a commercial species-adapted indirect CCHFV-IgG immunofluorescence assay (IFA) to obtain the result.
Samples collected from the field were transferred through a cold chain system and stored in a −80 °C freezer until RNA extraction. Total RNA for RT-PCR and real-time PCR was subsequently extracted and purified from frozen tissues using the Viral Nucleic Acid Isolation Kit (Silica-Based Spin Column) from Jiangsu Bioperfectus Technologies Co Ltd. (Jiangsu), following the manufacturer's protocols. This process aimed to eliminate genomic (g) DNA.
Detection of CCHFV RNA in real-time in ticks and sera
-
Serum samples and ticks were individually washed twice with PBS and crushed with a pestle in 200–300 µl of liquid nitrogen in 2 ml cryogenic vials to detect CCHFV RNA. RNA extraction was performed using the QIAamp Viral RNA Mini Kit according to the manufacturer's instructions, and total RNA was stored at −70 °C until use. Gel electrophoresis assessed RNA quality, where the presence of two distinct bands indicated high-quality RNA: the top band represented 28S ribosomal RNA (rRNA) at 4.8 kb, and the lower band represented 18S rRNA at 2.0 kb. Additionally, an in-house molecular method was used alongside a commercial kit for CCHF virus detection.
Questionnaire for data collection
-
A comprehensive questionnaire gathered socio-demographic data and assessed CCHF risk factors. Before administering the structured questionnaire, community engagement activities identified potential additional risk factors for CCHF exposure. Data collected from livestock owners included animal types and numbers, sample collection details, location, weather conditions, and individual animal specifics. Intrinsic factors (species, sex, age, and breed) and extrinsic factors (husbandry practices, body condition score, and tick infestation count) were recorded. Questions on CCHF awareness and public health aspects included closed, multiple-choice, and open-ended questions. Moderated interviews were conducted with farm owners in the local language.
Data analysis
-
All data underwent statistical analysis using SPSS Statistics 23.0. Proportions were calculated for qualitative variables, while mean with standard deviation (SD) and median with interquartile range (IQR) were calculated for quantitative variables. The chi-square test of independence and the Fisher exact test were utilized to determine associations among various independent factors (species, sex, breed, housing, hygiene, tick infestation, body condition score, and feeding systems) with CCHF seropositivity rates in cattle, sheep, camels, goats, and chickens. Minitab® 18 software was employed, with statistical significance set at p < 0.05[14].
-
Data extracted from Afghanistan's national surveillance system for 2007−2024 revealed 4,667 suspected cases, with 2651 laboratory-confirmed positives and 463 reported deaths. Additional cases were reported annually: 163 in 2016, 245 in 2017, 483 in 2018, 412 in 2022, 1,442 in 2023, and 113 as of March 2024, with the highest in 2023 (Fig. 1). Notably, confirmed positive cases peaked in 2023 (1,236), followed by 2022 (389), 2018 (139), and 2017 (104). This indicates an annual increase in CCHF cases, posing a significant public health threat, with a total case fatality rate of 463, highest in 2023 (114) (Fig. 1).
Figure 1.
Number of suspected and confirmed CCHF cases and death in Afghanistan, 2007–2024. The horizontal axis year (from 2007 to 2024) and vertical axis shows the number of CCHF cases.
From 2007 to 2024, the average case fatality ratio (CFR) of confirmed CCHF cases in Afghanistan was 30.2%. The CFR varied annually: 36% in 2016, 48% in 2017, 42.2% in 2018, 32% in 2019, 29% in 2020, 25% in 2021, 17% in 2022, 11% in 2023, and 2.1% as of March 2024. While CCHF cases increased until 2018, deaths subsequently declined (Fig. 1). Possible reasons for reduced CFR include improved public knowledge leading to prompt action, rapid blood donation supply, and increased preventive measures. Comparing January−March incidence from 2022−2024, no cases were reported in January−March 2022−2023, but 26 cases in January 2024, 47 in February, and 64 in March, indicating an anticipated increase in 2024 prevalence. Occupationally, most reported cases were in the 'others' category (23%), followed by unemployed (17%), housewives (14.5%), health staff (12.8%), shepherds (11%), butchers (7%), animal dealers and farmers (7.6%), and students (6.7%) (Fig. 2).
Figure 3.
National surveillance data due to CCHF outbreak on an annual basis for the northeast region provinces (Kunduz, Takhar, Badakhshan, and Baghlan) during the period of 2007−2024. The horizontal axis shows CCHF prevalence on year basis and vertical axis shows the number of CCHF confirmed cases by the national surveillance system for the northeast region provinces.
Prevalence of CCHF in the northeast during the years 2007−2024
-
Data from the national surveillance system for northeast region provinces (Kunduz, Takhar, Badakhshan, and Baghlan) showed higher CCHF prevalence in Kunduz (29.6%), followed by Takhar (25.4%), Badakhshan (24%), and Baghlan (20.8%) (Fig. 3). These findings suggest a higher likelihood of future prevalence in Kunduz and Takhar provinces. Hence, early mitigation is crucial, necessitating intensified biosecurity and tick prevention measures on animal farms.
Figure 2.
Occupational prevalence due to CCHF for the period of 2007−2024. The blue color indicates number of recorded cases while the dark blue color indicates the number of death cases respectively. The horizontal axis indicates the occupation of persons, and the vertical axis indicates the total number of CCHF cases due to occupational incidences of CCHF for the period of 2007−2024.
Ticks' identification
-
A total of 720 tick samples were collected, each containing an average of 27 ticks, totaling 4,672 ticks from Kunduz and Takhar provinces (Fig. 4). Tick species were identified based on morphological characteristics, revealing Hyalomma (H. asiaticum and H. marginatum), Rhipicephalus, Argas, Ornithodorus, Dermacentor, and Linognathus ticks. Hyalomma species were the most prevalent, followed by Rhipicephalus, while Dermacentor was least found. This indicates a significant presence of Hyalomma ticks, the primary vectors of CCHF, suggesting a high risk of transmission from infected animals to humans in the region and nationally (Fig. 4).
Figure 4.
Different species of ticks presents in Kunduz and Takhar provinces with their percentage. Each species of the ticks found are exhibited in the figure with number and percentage.
Laboratory investigations for CCHFV by RT-PCR and ELISA
-
A total of 720 ticks and 480 blood samples were collected, covering eight districts in two provinces equally. Among the samples tested by RT-PCR and IgG ELISA, 73 ticks in Kunduz and 81 ticks in Takhar were confirmed positive. In blood samples, 29 in Kunduz and 36 in Takhar tested positive (Fig. 5). Seropositivity was higher in Takhar than in Kunduz, with Rustaq in Takhar showing the highest prevalence. In Kunduz, Dasht-e-Archi had the highest prevalence. Overall, 102 ticks (17%) and 117 blood samples (19.5%) out of 720 and 480, respectively, were presumed positive for CCHF (Fig. 5).
Evaluation of intrinsic and extrinsic factors
-
Intrinsic factors, notably animal species, demonstrated a significant association with CCHF prevalence, with cattle showing the highest prevalence, followed by sheep, goats, and camels. Seroprevalence was notably higher in females than males (Table 1). Animals older than 2 years were more susceptible than younger ones, although differences between indigenous and exotic breeds were non-significant, despite higher prevalence in indigenous animals (Tables 2, 3). Extrinsic factors such as housing system, feeding, hygiene practices, body condition score, and tick infestation were also explored (Tables 4−8). Free-ranging animals had a higher prevalence than tethered ones, with significant associations observed between housing systems and seroprevalence (Table 4). Pasture-grazing animals exhibited higher seroprevalence than stall-fed ones, while animals receiving good hygienic practices had lower prevalence compared to those with poor hygiene (Tables 5, 6). Obese animals demonstrated a higher prevalence than emaciated and average-weight animals, with significant differences based on body condition scores (Table 7). Significant associations were found within districts and between provinces (Kunduz and Takhar) regarding tick infestation, with tick-infested animals showing higher seroprevalence (Tables 8, 9).
Table 1. Association of sera-molecular prevalence of CCHF within animal species in the Kunduz and Takhar provinces of Afghanistan.
Study province Study district Variables Examined Positive Seroprevalence (%) χ2 value p-value Kunduz Kunduz-Center Cattle 60 15 30 0.1816 0.0609 Sheep 40 9 32.5 Goat 30 3 26.66666667 Camel 15 0 13.33333333 Chicken 5 0 0 Dasht-e-Archi Cattle 60 18 25 0.6031 0.088 Sheep 40 13 22.5 Goat 30 8 10 Camel 15 2 0 Chicken 5 0 0 Imam Sahib Cattle 60 9 15 0.5714 0.061 Sheep 40 11 27.5 Goat 30 5 16.66666667 Camel 15 2 13.33333333 Chicken 5 0 0 Char Dara Cattle 60 5 8.333333333 0.0742 0.045 Sheep 40 4 10 Goat 30 3 10 Camel 15 0 0 Chicken 5 0 0 Takhar Taloqan Cattle 60 14 23.33333333 0.4867 0.067 Sheep 40 11 27.5 Goat 30 5 16.66666667 Camel 15 1 6.666666667 Chicken 5 0 0 Rustaq Cattle 60 22 36.66666667 0.5683 0.109 Sheep 40 16 40 Goat 30 9 30 Camel 15 3 20 Chicken 5 0 0 Khwaja Bahawodeen Cattle 60 9 15 0.4477 0.053 Sheep 40 8 20 Goat 30 4 13.33333333 Camel 15 0 0 Chicken 5 0 0 Khwaja Ghar Cattle 60 3 5 0.4953 0.043 Sheep 40 5 12.5 Goat 30 2 6.666666667 Camel 15 0 0 Chicken 5 0 0 Table 2. Association of sera-molecular prevalence of CCHF within sex of animals in the Kunduz and Takhar provinces of Afghanistan.
Study province Study district Variables Examined Positive Seroprevalence (%) χ2 value p-value Kunduz Kunduz-Center Male 77 12 15.58441558 0.5098 0.0361 Female 73 15 20.54794521 Dasht-e-Archi Male 77 11 14.28571429 0.0052 0.0302 Female 73 30 41.09589041 Imam Sahib Male 77 10 12.98701299 0.1713 0.0103 Female 73 17 23.28767123 Char Dara Male 77 4 5.194805195 0.2301 0.0016 Female 73 8 10.95890411 Takhar Taloqan Male 77 11 14.28571429 0.1079 0.0067 Female 73 20 27.39726027 Rustaq Male 77 19 24.67532468 8.125 0.015 Female 73 31 42.46575342 Khwaja Bahawodeen Male 77 7 9.090909091 0.1222 0.003 Female 73 14 19.17808219 Khwaja Ghar Male 77 3 3.896103896 0.1914 0.001 Female 73 7 9.589041096 Table 3. Association of sera-molecular prevalence of CCHF within age of animals in the Kunduz and Takhar provinces of Afghanistan.
Study province Study district Variables Examined Positive Seroprevalence (%) χ2 value p-value Kunduz Kunduz-Center < 6 months 20 3 15 1 0.0137 1 > Year 30 3 23.33333333 > 2 Year 40 7 25 2 > Year 60 14 35 Dasht-e-Archi < 6 months 20 3 15 1 0.027 1 > Year 30 7 10 > 2 Year 40 10 17.5 2 > Year 60 21 23.33333333 Imam Sahib < 6 months 20 2 10 1 0.012 1 > Year 30 5 16.66666667 > 2 Year 40 9 22.5 2 > Year 60 11 18.33333333 Char Dara < 6 months 20 1 5 1 0.007 1 > Year 30 3 10 > 2 Year 40 2 5 2 > Year 60 6 10 Takhar Taloqan < 6 months 20 2 10 1 0.016 1 > Year 30 5 16.66666667 > 2 Year 40 9 22.5 2 > Year 60 15 25 Rustaq < 6 months 20 5 25 1 0.035 1 > Year 30 10 33.33333333 > 2 Year 40 13 32.5 2 > Year 60 22 36.66666667 Khwaja Bahawodeen < 6 months 20 2 10 1 0.0103 1 > Year 30 3 10 > 2 Year 40 5 12.5 2 > Year 60 11 18.33333333 Khwaja Ghar < 6 months 20 1 5 1 0.006 1 > Year 30 2 6.666666667 > 2 Year 40 2 5 2 > Year 60 5 8.333333333 Table 4. Association of sera-molecular prevalence of CCHF within breed of animals in the Kunduz and Takhar provinces of Afghanistan.
Study province Study district Variables Examined Positive Seroprevalence (%) χ2 value p-value Kunduz Kunduz-Center Indigenous 140 26 18.57142857 0.5571 0.45 Exotic 10 1 10 Dasht-e-Archi Indigenous 140 39 27.85714286 0.6757 0.504 Exotic 10 2 20 Imam Sahib Indigenous 140 26 18.57142857 0.5571 0.45 Exotic 10 1 10 Char Dara Indigenous 140 12 8.571428571 0.3558 0.401 Exotic 10 0 0 Takhar Taloqan Indigenous 140 30 21.42857143 0.4654 0.465 Exotic 10 1 10 Rustaq Indigenous 140 47 33.57142857 0.8684 0.541 Exotic 10 3 30 Khwaja Bahawodeen Indigenous 140 20 14.28571429 0.7389 0.429 Exotic 10 1 10 Khwaja Ghar Indigenous 140 10 7.142857143 0.399 0.395 Exotic 10 0 0 Table 5. Association of sera-molecular prevalence of CCHF with housing system of animals in the Kunduz and Takhar provinces of Afghanistan.
Study province Study district Variables Examined Positive Seroprevalence (%) χ2 value p-value Kunduz Kunduz-Center Extensive 75 19 25.33333333 0.5086 0.007 Intensive 75 8 10.66666667 Dasht-e-Archi Extensive 75 29 38.66666667 0.0181 0.023 Intensive 75 12 16 Imam Sahib Extensive 75 22 29.33333333 0.0031 0.018 Intensive 75 5 6.666666667 Char Dara Extensive 75 10 13.33333333 0.026 0.003 Intensive 75 2 2.666666667 Takhar Taloqan Extensive 75 26 34.66666667 0.0005 0.029 Intensive 75 5 6.666666667 Rustaq Extensive 75 39 52 0.0005 0.07 Intensive 75 11 14.66666667 Khwaja Bahawodeen Extensive 75 17 22.66666667 0.0007 0.01 Intensive 75 4 5.333333333 Khwaja Ghar Extensive 75 9 12 0.0141 0.003 Intensive 75 1 1.333333333 Table 6. Association of sera-molecular prevalence of CCHF with feeding system of animals in the Kunduz and Takhar provinces of Afghanistan.
Study province Study district Variables Examined Positive Seroprevalence (%) χ2 value p-value Kunduz Kunduz-Center Stall feeding 75 6 8 0.0076 0.0145 Pasture grazing 75 21 28 Dasht-e-Archi Stall feeding 75 6 8 4.8928 0.064 Pasture grazing 75 35 46.66666667 Imam Sahib Stall feeding 75 3 4 0.0001 0.027 Pasture grazing 75 24 32 Char Dara Stall feeding 75 1 1.333333333 0.0053 0.005 Pasture grazing 75 11 14.66666667 Takhar Taloqan Stall feeding 75 5 6.666666667 0.0004 0.029 Pasture grazing 75 26 34.66666667 Rustaq Stall feeding 75 9 12 0.002 0.088 Pasture grazing 75 41 54.66666667 Khwaja Bahawodeen Stall feeding 75 3 4 0.002 0.013 Pasture grazing 75 18 24 Khwaja Ghar Stall feeding 75 2 2.666666667 0.066 0.001 Pasture grazing 75 8 10.66666667 Table 7. Association of sera-molecular prevalence of CCHF with hygenenic measures for animals in the Kunduz and Takhar provinces of Afghanistan.
Study province Study district Variables Examined Positive Seroprevalence (%) χ2 value p-value Kunduz Kunduz-Center Good 75 5 6.666666667 0.0024 0.018 Poor 75 22 29.33333333 Dasht-e-Archi Good 75 7 9.333333333 0.0001 0.056 Poor 75 34 45.33333333 Imam Sahib Good 75 4 5.333333333 0.0007 0.023 Poor 75 23 30.66666667 Char Dara Good 75 2 2.666666667 0.026 0.003 Poor 75 10 13.33333333 Takhar Taloqan Good 75 7 9.333333333 0.0052 0.019 Poor 75 24 32 Rustaq Good 75 12 16 0.0013 0.061 Poor 75 38 50.66666667 Khwaja Bahawodeen Good 75 4 5.333333333 0.0077 0.01 Poor 75 17 22.66666667 Khwaja Ghar Good 75 3 4 0.2205 0.0008 Poor 75 7 9.333333333 Table 8. Association of sera-molecular prevalence of CCHF with body condition score of animals in the Kunduz and Takhar provinces of Afghanistan.
Study province Study district Variables Examined Positive Seroprevalence (%) χ2 value p-value Kunduz Kunduz-Center Obese 50 15 44 5.9152 0.0002 Average 50 4 14 Emaciated 50 8 24 Dasht-e-Archi Obese 50 22 30 1.0036 0.001 Average 50 7 8 Emaciated 50 12 16 Imam Sahib Obese 50 16 32 1.3038 0.0004 Average 50 3 6 Emaciated 50 8 16 Char Dara Obese 50 7 14 7.6074 1.194 Average 50 1 2 Emaciated 50 4 8 Takhar Taloqan Obese 50 16 32 2.748 0.0003 Average 50 4 8 Emaciated 50 11 22 Rustaq Obese 50 17 34 2.386 0.0053 Average 50 6 12 Emaciated 50 27 54 Khwaja Bahawodeen Obese 50 11 22 2.4731 0.0001 Average 50 1 2 Emaciated 50 9 18 Khwaja Ghar Obese 50 4 8 0.0001 2.627 Average 50 1 2 Emaciated 50 5 10 Table 9. Association of sera-molecular prevalence of CCHF with tick infestation in animals in the Kunduz and Takhar provinces of Afghanistan.
Study province Study district Variables Examined Positive Seroprevalence (%) χ2 value p-value Kunduz Kunduz-Center Indigenous 75 19 25.33333333 0.0508 0.007 Exotic 75 8 10.66666667 Dasht-e-Archi Indigenous 75 32 42.66666667 0.0013 0.041 Exotic 75 9 12 Imam Sahib Indigenous 75 18 24 0.1103 0.005 Exotic 75 9 12 Char Dara Indigenous 75 8 10.66666667 0.2663 0.0008 Exotic 75 4 5.333333333 Takhar Taloqan Indigenous 75 24 32 0.0052 0.019 Exotic 75 7 9.333333333 Rustaq Indigenous 75 39 52 0.0005 0.0702 Exotic 75 11 14.66666667 Khwaja Bahawodeen Indigenous 75 18 24 0.002 0.013 Exotic 75 3 4 Khwaja Ghar Indigenous 75 8 10.66666667 0.0666 0.0018 Exotic 75 2 2.666666667 -
Afghanistan is currently facing an intensified surge of Crimean-Congo Hemorrhagic Fever (CCHF) nationwide. Domestic ruminants, including cattle, sheep, goats, camels, and chickens, can act as reservoir hosts for CCHFV, aiding virus transmission through tick bites or direct contact with infected tissues. This situation raises substantial public health concerns. From 2007 to 2024, Afghanistan has seen an annual rise in confirmed CCHF cases and associated deaths. Public surveillance data indicates 4,667 suspected cases during this period, with 2,651 confirmed positive cases and 463 deaths. Specific numbers for certain years include: 163 cases in 2016, 245 in 2017, 483 in 2018, 412 in 2022, 1,442 in 2023, and 113 as of March 2024. The highest confirmed cases were in 2023 (1,236), followed by 2022, 2018, and 2017. Despite a rise until 2018, there has been a decline in deaths since then[5].
The present investigation compared CCHF incidences nationally from January to March in 2022 to 2024. Surprisingly, no cases were reported in January to March in 2022 and 2023. However, in January 2024, 26 cases were confirmed, followed by 47 in February and 64 in March, totaling 137 cases with a CFR of 1%. These findings indicate a higher tendency for increased CCHF cases in 2024 compared to previous years[15].
The present findings on occupational transmission of CCHF from 2007 to 2024 aligns with previous studies[16]. Most cases were from individuals categorized as 'others' (23%), followed by the unemployed (17%), housewives (14.5%), health staff (12.8%), shepherds (11%), butchers (7%), animal dealers, and farmers (7.6%), and students (6.7%). These patterns correspond with studies by Ahmad et al., Sahak, and research in Pakistan, which reported CFR rates ranging from 10% to 40%[5,17].
Program experts suggest that the increase in CCHF cases may be linked to environmental factors. Drought and a lack of fodder in the West and North regions have led to dry pastures, prompting the migration of livestock and people to areas with better grazing conditions. This movement increases the potential for infected tick exposure as migrating herds mix with others[2,4,10].
Data from the national surveillance system for the northeast region provinces (Kunduz, Takhar, Badakhshan, and Baghlan) showed Kunduz had the highest prevalence (29.6%), followed by Takhar (25.4%), Badakhshan (24%), and Baghlan (20.8%)[18]. This suggests a higher likelihood of prevalence in Kunduz and Takhar in the future. Domestic ruminants like cattle, goats, and sheep can act as reservoir hosts for CCHFV, making tick-borne diseases a significant concern due to their veterinary and public health implications. Hyalomma species are major vectors for CCHFV transmission to both animal and human hosts through bites[18,19]. Across eight districts in the Kunduz and Takhar provinces, a total of 720 ticks and 480 blood samples were collected. Of the 360 ticks sampled in each province, 73 in Kunduz and 81 in Takhar tested positive for CCHFV using RT-PCR and IgG ELISA (Fig. 5). Regarding blood samples, 29 out of 240 were positive in Kunduz, while 36 out of 240 were positive in Takhar. Seropositivity was higher in Takhar province than in Kunduz. In Takhar, Rustaq had the highest prevalence, followed by Taloqan, Khwaja Bahawodeen, and Khwaja Ghar, ranging from 10% to 2%. In Kunduz, Dasht-e-Archi had the highest prevalence, followed by Kunduz Center, Imam Sahib, and Char Dara, ranging from 8.2% to 2.4% (Fig. 5).
Remarkably, among the eight districts of both provinces, Rustaq showed the highest prevalence of CCHF at 10%, followed by Dasht-e-Archi at 8.2%. Across both provinces, 102 (17%) tick samples were presumed positive and 117 (19.5%) blood samples out of 720 and 480, respectively (Fig. 5).
These findings are in line with parallel studies conducted in various countries. For instance, in Gambia[20], a higher prevalence was reported in cattle compared to small ruminants (sheep and goats), which aligns with the present results. Similarly, studies[18,21] in different locations also revealed higher seropositivity of CCHFV in cattle than in goats and sheep, consistent with the present findings. Additionally, research in Pakistan reported the highest seroprevalence of CCHFV antibodies in cattle, followed by sheep and goats. Studies in Corsica, France[22] and Kosovo, Germany, also found higher seropositivity in cattle compared to sheep and goats[23].
The present findings reveal higher seroprevalence in cattle compared to sheep, goats, and camels, suggesting they could serve as a source of CCHFV transmission to these animals during grazing interactions. This possibility is supported by previous research[24]. The elevated seroprevalence in cattle may be attributed to Hyalomma ticks, the primary carriers of CCHFV, which prefer feeding on larger animals like cattle. Ticks readily attach to cattle for feeding, facilitating efficient viral transfer between infected ticks and cattle. CCHFV replicates to higher levels in cattle compared to sheep, goats, and camels, leading to a higher viral load in the bloodstream. This increases the likelihood of ticks acquiring CCHFV when feeding on infected cattle.
In the present study, a significantly higher seroprevalence of CCHF was found in female domestic animals compared to males (p > 0.05). This aligns with previous research[18,20,25,26]. The elevated seroprevalence in female domestic animals could be attributed to factors such as pregnancy stress, lactation stress, and limited access to balanced nutrition, which may reduce immunity and decrease their resistance to tick infestations.
The present study supports previous findings that local breeds exhibit higher seropositivity compared to exotic breeds, as reported in previous studies[18,25]. Similarly, previous research[18,25] indicates that indigenous cattle breeds experience more tick infestations and external parasites compared to exotic breeds, potentially leading to higher seroprevalence. This similarity could be attributed to factors such as poor hygiene, limited access to quality feed, and inferior husbandry practices observed in Indigenous breeds compared to exotic breeds found in the study areas. The current study highlights higher seroprevalence in animals raised extensively or on communal grazing systems, while those in intensive housing systems exhibit lower seroprevalence. These findings align with previous research[18,24,27] . The increased seroprevalence in extensively raised animals may be attributed to their closer proximity to tick vectors, lack of acaricide use, and poor hygiene management practices on the farm. Conversely, the lower seroprevalence in animals kept in intensive housing systems may result from effective tick control measures, such as regular acaricide application and good hygiene practices, which reduce tick populations[27].
Early studies support the present findings that higher seroprevalence in older and tick-infested cattle is age-dependent[28]. Seroprevalence in cattle increases with age and the presence of tick infestation, as documented in previous research[29]. Studies conducted in Kenya, northwestern Senegal, Afghanistan, and Uganda[30] also support this association between seroprevalence and age in cattle. Additionally, research suggests that the seroprevalence of CCHFV antibodies in domestic ruminants is dependent on age, with older animals exhibiting higher seroprevalence rates than younger ones[21]. This higher seroprevalence with age may be attributed to increased production of IgG antibodies in response to continuous exposure to CCHFV-infected ticks in older animals in endemic areas, compared to younger animals with maternal immunity.
The present investigations have identified a correlation between the body condition of domestic animals and CCHFV antibody seroprevalence. Previous research[18] found a high seroprevalence in overweight ruminants, correlating with weight. They observed that obese animals were more susceptible to CCHF compared to emaciated animals due to weakened immunity. The present study supports this, revealing that obese domestic animals exhibited the highest seroprevalence, followed by those of average weight, and then emaciated animals, respectively.
Furthermore, heavily infested ruminants play a crucial role in CCHFV transmission and can become sources of infection for healthy animals compared to tick-free animals, as reported previously[18,31]. Similarly, it was reported that tick-infested cattle have a higher seroprevalence compared to tick-free animals[28], which is fully consistent with the current study.
Afghanistan, situated in the ecological range of the Hyalomma tick, experiences an annual increase in CCHF incidence[10,32]. The variation in seropositivity observed in the present study may be attributed to the endemicity of CCHF in the region, the significant abundance of ticks, and host behavior patterns influenced by climate changes and drought. Additionally, differences in laboratory examinations for molecular and serological detection of CCHFV antibodies, including specificity and sensitivity could contribute to these variations. These insights call for further investigation into the associated factors contributing to the rising number of CCHF cases within the country.
-
The higher seroprevalence underscores a significant healthcare concern, given the recent rise in CCHF cases and fatalities in Afghanistan. The initial report highlights a notably elevated prevalence of CCHFV nationally and regionally, urging urgent attention to mitigate further spread, particularly in livestock. Extrinsic risk factors (husbandry practices, animal condition, and tick infestation) and intrinsic factors (species, sex, age, and breed) show significant associations with CCHFV seroprevalence, detected through IgG antibodies and RT-PCR analysis. Collaborating with Afghan molecular experts, an in-house molecular method has been developed for CCHF virus detection in ticks and blood samples, facilitating deeper genome studies. Early detection and understanding of risk factors in animal hosts aid in mapping endemic areas. Given CCHF's impact on human health, especially those in direct animal contact, control strategies are imperative. Livestock plays a vital role in rural Afghans' livelihoods and can transmit diseases. Raising local awareness, collaborating with health and veterinary departments, promoting animal health practices, and intensifying livestock husbandry alongside establishing active disease surveillance are essential for enhancing one-health approaches.
-
All procedures were reviewed and approved by the Animal Care and Research Committee of Ministry of Agriculture Irrigation and Livestock (MAIL), identification number: (KBL-2023–MAIL-03), approval date: 2023-12-09, and implemented based on the standard of Experimental Animal Care and Use Guidelines of Animals. The research followed the "Replacement, Reduction, and Refinement" principles to minimize harm to animals. This article provides details on the housing conditions, care, and pain management for the animals, ensuring that the impact on the animals is minimized during the experiment.
-
The authors confirm contribution to the paper as follows: writing – draft manuscript preparation, investigation, conceptualization: Hamdard E; formal analysis, data curation: Karwand B, Din Muhammad S; data collection – review & editing, methodology: Zahir A, Din Muhammad S, Mosavi SH. writing – final draft and editing: Sayedpoor S. All authors reviewed the results and approved the final version of the manuscript.
-
The original contributions presented in this study are included in the article/supplementary material. Further inquiries can be directed to the corresponding author.
-
The authors declare that they have no conflict of interest.
- Copyright: © 2024 by the author(s). Published by Maximum Academic Press on behalf of Nanjing Agricultural University. This article is an open access article distributed under Creative Commons Attribution License (CC BY 4.0), visit https://creativecommons.org/licenses/by/4.0/.
-
About this article
Cite this article
Hamdard E, Zahir A, Mosawi SH, Din Muhammad S, Karwand B, et al. 2024. Descriptive epidemiology and seroprevalence investigations of Crimean-Congo Hemorrhagic Fever virus in domestic animals of northeast Afghanistan. Animal Advances 1: e007 doi: 10.48130/animadv-0024-0007
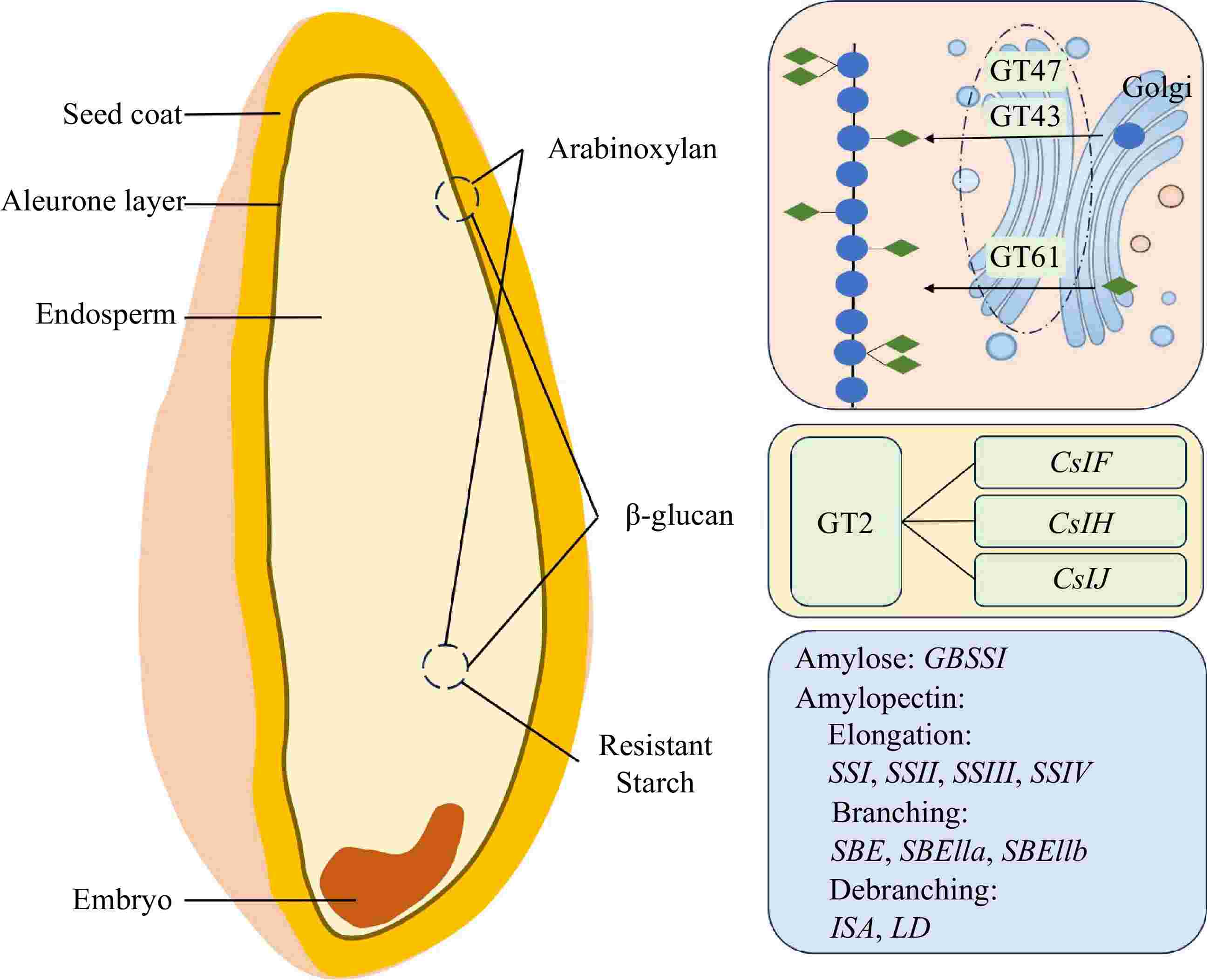