-
Orchardgrass (Dactylis glomerata L.) is an excellent cool season perennial grass that is widely cultivated around the world and it is the fourth most crucial forage grass[1−3]. Because of its strong adaptability, rich nutrition, large biomass, and soft grass quality, orchardgrass is widely used in grazing and for the production of hay or silage[4,5]. In addition, it also plays an important role in ecological remediation and grassland construction, such as stone desertification control and returning farmland to grass[6]. As a material basis for reproduction and application in improving degraded grassland and planting artificial grassland, seed is critical in grassland construction and economic development of animal husbandry[7]. However, lodging can seriously affect seed production when it encounters wind and rain during the filling stage due to the large biomass and softness of orchardgrass[8].
Seed yield is an important characteristic of forage and turf grass, thus seed multiplication is economically relevant for novel grass cultivars to compete commercially[9]. In recent years, seed production had induced wide concern for the breeders and producers. The yield of forage seeds is compared with crop seeds, which is usually very low and variable, only 10%−20% of the above-ground matter harvested as seed[10]. Grass seed yield is a complex trait which is affected by genetic properties, cultivation management as well as environmental factors[11]. Cultivation management could effectively optimize seed yield by establishment techniques, the use of plant growth regulators, fertilization, harvesting, and seed cleaning[9].
Plant growth regulators (PGRs) are synthetic compounds that have similar effects to natural plant hormones and are recognized as exogenous non-nutritive chemicals for target plants. As a growth blocker, Chlorocholine chloride (CCC) (also called Chlormequat chloride) is an excellent PGR to reduce reproductive stem length and lodging, make the leaves green and thicker, increase the chlorophyll content, and develop the root system. CCC blocks the biosynthesis of endogenous gibberellin (GA), thus reducing cell division in the internode region without affecting the division of apical meristem and delaying cell elongation and plant dwarfing[12,13]. CCC has been recommended to be applied to reduce lodging in seed production[14]. Stahli et al. also found that CCC could improve lodging resistance and increase Triticum aestivum grain yield[15,16]. Trinexapac-ethyl (TE) is also a plant growth regulator and has been widely adopted as a lodging control agent in forage and turf grass seed production[17]. It controls the growth of plants by reducing the content of GA, which is similar to CCC. TE also controls the height of plants when the crop is growing vigorously, thereby increasing seed yield[18]. TE-induced increases in seed yield were related to improvements in floret production and seed set[19]. The increase in seed yield had been reported in a lot of grass species by the use of chemical PGRs[9]. To date, the fertilization, cultivation mode, and application of PGRs have been proven to increase the number of inflorescence[20], the number of seeds per panicle[21], and the number of seeds[22]. Those seed production factors in orchardgrass directly or indirectly improve the yield of orchardgrass seed.
Currently, there is a large demand for orchardgrass seeds. Besides the improvement of traditional cultivation and fertilization measures, it is also crucial to increase seed yield through the use of plant exogenous hormones. This study aimed to explore the effects of various plant growth regulators and their combinations on reproductive traits, seed yield components, and cell level of orchardgrass. Thus, the effects of different plant growth regulators on orchardgrass seed yield, traits, and microstructure cells, and the most suitable treatment method for the production of their seeds were studied and then incorporated into production to help increase the yield of orchardgrass seed.
-
The plant material used in this experiment was a nationally-approved variety 'Dianbei' (Dactylis glomerata cv. 'Dianbei') selected by Sichuan Agricultural University, with good palatability, well-adapted, high nutritional value, and high yield. The experiment was carried out at the experimental site (E103°39', N30°33') of the modern agricultural base of Sichuan Agricultural University (Chengdu, China) for two years. The experimental site was in the subtropical humid monsoon climate zone, with an altitude of 514 m. The soil was purple loam soil with a pH of 6.2. The soil available phosphorus content was 1.81 mg·kg−1, available nitrogen content was 52 mg·kg−1, and available potassium content was 79 mg·kg−1. Before sowing, 20,000 kg·hm−2 of organic fertilizer was applied to the test site and topdressing was applied during tillering and jointing. After removal of weeds and debris for one week, 'Dianbei' was sown on September 15, 2016. The sowing density was equivalent to 20 kg·hm−2. By using drill seeding, 3 m × 5 m of the plot area was prepared, with row spacing at 50 cm. The protection lines were set at 3 m around the whole test site, whereas the spacing between plots was 1 m. Throughout the entire growth observation period, tilling and weed removal were carried out from time to time to prevent diseases and insect pests.
Treatments
-
The trial was designed in a single-factor randomized plot with three replicates. The growth period of this experiment was based on the expression of Zadoks et al.[23]. Within the period of GS32 (jointing stage: two nodes), foliar sprays were applied to 'Dianbei' at different concentrations, followed by second spray after two weeks. Tap water acted as a control (CK) in this study.
The concentrations of plant growth regulators were determined based on the results from previous studies[24,25], and the plot experiment which was conducted in the early stage of the experiment. The dosage of each plant growth regulator is listed in Table 1.
Table 1. Experiment application rate.
Treatment Serial number Application rate in 2017 and 2018
at the stage of GS32 (g·hm−2)Chlorocholine chloride (A) A1 500 A2 750 A3 1,000 A4 1,250 Trinexapac-ethyl (B) B1 100 B2 200 B3 300 B4 400 Chlorocholine chloride & Trinexapac-ethyl (C) C1 500 + 50 C2 500 + 150 C3 1,000 + 50 C4 1,000 + 150 Plant measurements
-
A few measurements were taken at the mature stage of orchardgrass, including plant height, internode number, the length and stem diameter of the second internode, the length and width of the flag leaf, and the length and width of the top second leaf. During the flowering period, a 1 m2 sample (excluding marginal rows) was randomly selected from each experimental plot to determine the number of fertile tillers of orchardgrass. The procedures were repeated four times for each plot. Twenty fertile tillers were randomly selected from each plot to determine the number of spikelets per fertile tiller, whereas 20 spikelets were randomly selected from each plot to determine the number of florets per spikelet. The theoretical seed yield (
) was calculated. Harvested seeds were weighed after drying, threshing, and cleaning. After that, the number of seeds with a caryopsis was counted, and the number of these seeds was divided by the number of flowers per spikelet to calculate the seed-setting rate. All the measuring indexes are shown in Table 2.fertiletillersUnitarea×spikeletfertiletillers×floretsspikelet×averageseedweight Table 2. Total measurement index.
Morphological character Reproductive character Plant height, PH (cm) The number of fertile, NFT (tillers·m−2) Internode number, IN Spikes per fertile tillers, SFT Internode length, IL (cm) Florets per spikelet, FS Stem diameter, SD (mm) Seed setting rate, SSR (%) Flag leaf width, FLW (mm) 1000-seed weight, TSW (g) Flag leaf length, FLL (cm) Actual seed yield, ASY (kg·hm−2) Width of top second leaf,
WTSL (mm)Theoretical seed yield, TSY (kg·hm−2) Length of top second leaf,
LTSL (cm)The histological analysis of vascular bundle cells was carried out by selecting the cross-section of the middle stem of the second segment of the stem base. After that, the plant cells were stained with Safranin O-Fast Green Stain, followed by observation of the sclerenchyma and xylem cells under the electron microscope at 400 x.
Statistical analysis
-
All the experimental data were summarized using Microsoft Excel 2016. To investigate the effects of different PGRs on the seed yield and related traits of orchardgrass, One-Way ANOVA was used in SPSS Statistics 27 (SPSS Inc., New York, USA) to conduct a single factor variance analysis for each indicator. The level of significant difference was p < 0.05. LSD tests were used to compare the levels of each indicator for two years. The correlation heat map visualization (Pearson) and principal component analysis of orchardgrass were analyzed by Origin 2024 (Origin Lab Corp Inc., USA).
-
After treatment with different concentrations of CCC and TE, the plant height of orchardgrass was significantly reduced, and the effect was more significant with the increased concentration (p < 0.05). The application of CCC with a dosage of 500 g·hm−2 inhibited the internode number, and the inhibitory effect decreased with the increase of CCC concentration, showing low promotion and high inhibition. The internode number was significantly reduced for treatment TE alone and a CCC + TE mixture compared with the control. After the application of CCC, internode length was inhibited, and with the increase in concentration, the internode length became shorter. When TE and CCC + TE were combined, a low concentration had a significantly promoting effect on internode length, and with the increase in concentration, the internode length of D. glomerata was first increased and then decreased. CCC significantly increased the stem diameter of orchardgrass at 750 g·hm−2, and the stem diameter was decreased by TE treatment at all concentrations. Under the mixed application of CCC + TE, with the concentration at 500 + 50 g·hm−2, the stem diameter was the largest. However, the stem diameter was reduced when the concentration was too high (Table 3).
Table 3. Effects of plant growth regulators on the morphology characters of Dactylis glomerata in 2017−2018.
PGR No. Plant height (cm) Internode number Internode length (cm) Stem diameter (mm) Flag leaf width (mm) Flag leaf length (cm) Width of top
second leaf (mm)Length of top second leaf (cm) A CK 129.9a 4.58d 14.90a 5.18b 7.82b 31.42a 7.96a 39.34a A1 119.6b 4.22e 12.12b 4.75c 7.66c 29.20b 7.35c 36.72b A2 106.7c 4.81c 12.00bc 5.30a 7.84b 31.56a 7.68b 36.12bc A3 98.6d 5.25b 10.20c 4.92b 8.01a 27.65b 7.92a 33.40c A4 94.0d 5.52a 9.40c 4.69c 8.13a 28.66b 7.94a 34.29c B CK 129.9a 4.58a 14.90b 5.18a 7.82bc 31.42a 7.96d 39.34ab B1 119.8b 4.25bc 15.43a 4.98b 7.67c 31.02a 7.87d 40.00a B2 116.0c 4.30b 14.98b 4.68c 8.28a 30.03ab 8.61a 36.96c B3 111.6d 4.26bc 13.63c 5.15a 7.99b 27.99c 8.15c 36.23c B4 104.2e 3.91c 13.62c 4.87b 8.34a 29.31b 8.51b 35.70c C CK 129.9a 4.58a 14.90b 5.18b 7.82bc 31.42a 7.96b 39.34a C1 118.9b 4.06b 15.61a 5.39a 8.41a 30.33b 8.39a 37.85b C2 119.1b 4.07b 14.65b 4.79c 7.46d 25.93d 7.67c 34.72c C3 110.8c 3.71d 13.97bc 4.72d 7.78c 31.10a 7.87b 37.61b C4 114.0c 3.83c 13.60bc 4.79d 7.92b 28.13c 8.43a 35.15c Different letters in the same column indicate significant differences at the p < 0. 05 level. When high concentrations of CCC above 750 g·hm−2 and over 200 g·hm−2 of TE were applied, the width of the flag leaf was increased. Under a mixture of CCC + TE at 500 + 50 g·hm−2, the flag leaf width was the largest, which was 8.41 mm. On the contrary, except for the mixed application of CCC + TE at 500 + 50 and 1,000 + 150 g·hm−2, other concentrations inhibited the width of the flag leaf. The length of the flag leaf decreased with increasing concentrations of TE. However, the CCC alone and the mixed application of CCC + TE showed a trend of decreasing and then increasing with the increase in concentration. The variation of the width and length of the top second leaf was similar to the width and length of flag leaf (Table 3).
In terms of the effects of two PGRs on the morphological characters of orchardgrass, the present study showed that the high concentration of CCC alone (1,250 g·hm−2) had the lowest plant height, which was 94.0 cm, whereas the shortest internode length was 9.40 cm. Application of TE alone had the greatest effect on the length and width of the top second leaf. At a TE concentration of 200 g·hm−2, the largest width of the top second leaf was 8.61 mm. On the other hand, the longest length of the second to top leaf was 40 cm when the concentration was 100 g·hm−2. Mixed application of CCC + TE had the greatest effects on internode number, stem diameter, and flag leaf width. When the concentration was 1,000 + 50 g·hm−2, the minimum internode number was 3.71. At a concentration of 500 + 50 g·hm−2, the maximum stem diameter and flag leaf width were 5.39 and 8.41 mm respectively among all treatments.
Effects of plant growth regulators on seed yield and yield component of Dactylis glomerata
-
According to the average data from a previous study in 2017−2018, CCC significantly increased the number of fertile tillers·m−2, but when the concentration reached 1,250 g·hm−2, the number of fertile tillers·m−2 decreased. The mixed application of different concentrations of TE, CCC + TE significantly increased the number of fertile tillers·m−2. The number of spikelets increased significantly under certain concentration conditions of PGRs, except for the concentration above 1,000 + 150 g·hm−2 under the mixed application of CCC + TE and when the concentration of TE was over 200 g·hm−2. PGRs increased the number of florets and the seed setting rate. When the concentration of CCC was 750 g·hm−2 and the concentration of TE was 100 g·hm−2, the 1000-grain weight of D. glomerata seeds was significantly increased compared with the control. For CCC + TE mixed treatments, the concentration of 500 + 50 g·hm−2 and 1,000 + 50 g·hm−2 significantly increased the 1000-grain weight of seeds. When the concentration of CCC was lower than 1,250 g·hm−2, the actual seed yield and theoretical yield of D. glomerata were significantly increased (p < 0.05). The actual seed yield and the theoretical yield increased with different concentrations of TE, except for the TE concentration at 400 g·hm−2, which was lower than other concentrations in the actual seed yield. For the combined application of CCC + TE, the actual and theoretical seed yields of all treatments were significantly higher than the control (p < 0.05) (Table 4).
Table 4. Effects of PGRs on seed yield and yield characters of Dactylis glomerata L. in 2017−2018.
PGR No. The number of fertile (tillers·m−2) Spikes per fertile tillers Florets per spikelet Seed setting rate (%) 1000-grain weight (g) Actual seed yield (kg·hm−2) Theoretical seed yield (kg·hm−2) A CK 116.50d 366.28a 4.01c 45d 0.62b 216c 1,057d A1 150.165b 365.57a 4.29a 53b 0.61b 253a 1,443b A2 167.25a 367.19a 4.11bc 55a 0.64a 248a 1,601a A3 132.75c 353.35b 4.13b 52bc 0.61b 222b 1,194c A4 89.42e 350.16b 4.15b 50c 0.63ab 186d 823e B CK 116.50d 366.44c 4.01c 45c 0.62b 216d 1,057e B1 141.67bc 449.22a 4.18b 52b 0.64a 278b 1,690b B2 163.59a 424.595b 4.27a 55a 0.62b 297a 1,820a B3 146.25b 355.58d 4.43a 53ab 0.62b 240c 1,412c B4 131.42c 359.17d 4.35a 45c 0.63b 209d 1,270d C CK 116.5e 366.44d 4.01b 45d 0.62b 216d 1,057e C1 135.17c 432.67c 4.06b 61b 0.66a 270bc 1,561c C2 147.34b 443.33b 4.42a 63ab 0.61b 320a 1,783b C3 159.42a 485.24a 4.12b 66a 0.65a 280b 2,068a C4 132.58d 362.69e 4.51a 53c 0.62b 256c 1,322d Different letters in the same column indicate significant differences at the p < 0.05 level. In terms of the effects of two PGRs on seed yield and yield component of orchardgrass, it was found that the high concentration of CCC alone was sprayed (1,250 g·hm−2), the lowest number of fertile tillers·m−2 was 89.42, while the lowest actual seed yield and theoretical yield were 186 kg·hm−2, and 823 kg·hm−2 respectively. The mixed application of CCC + TE had the greatest effects on actual seed yield at 500 + 150 g·hm−2, making the actual seed yield 320 kg·hm−2.
Correlation between the morphological and reproductive traits of Dactylis glomerata
-
The correlation analysis showed that the correlations among the morphological traits were not consistent under the different PGRs. Among them, under the CK, the number of internodes, the width of the flag leaf, and the length of the top second leaf were significantly negatively correlated, the length of the internode and the length of the top second leaf were also negatively correlated (Fig. 1a). After the treatment of CCC, the plant height of orchardgrass was extremely negatively correlated with the number of internodes, the width of the flag leaf, and the width of the top second leaf, but significantly positively correlated with internode length and the length of the top second leaf. The actual seed yield was not significantly correlated with plant height, but positively correlated with internode length, stem diameter, and the length of the top second leaf (Fig. 1b). Under TE treatment, plant height was extremely positively correlated with internode length, internode number, and the length of the top second leaf, but significantly negatively correlated with the flag leaf width. Actual seed yield was extremely positively correlated with plant height, internode number, and length. The length of the top second leaf was positively correlated (Fig. 1c). Under the combination of CCC + TE, the actual seed yield was significantly negatively correlated with the width of the flag leaf, and extremely negatively correlated with the length of the top second leaf (Fig. 1d).
Figure 1.
Correlation between the morphological traits of Dactylis glomerata. The correlation heat map visualization of (a) control, (b) CCC, (c) TE, and (d) CCC + TE are presented. ** significant correlation at the 0.01 level; * significant correlation at the level of 0.05.
The correlation analysis of reproductive traits of orchardgrass showed that under control treatment, the correlation coefficient between the actual seed yield and the number of fertile tillers·m−2 was −0.69, showing a significant negative correlation (Fig. 2a), but under treatment of CCC, the correlation coefficient was 0.95, showing a significant positive correlation (Fig. 2b). Under treatment of TE, there was a significant positive correlation between the actual seed yield and the number of fertile tillers·m−2, spikes per fertile tiller, and seed setting rate (Fig. 2c). Under the mixed treatment of CCC and TE, the actual seed yield was positively significant correlated with the seed setting rate, while the theoretical seed yield was extremely positively correlated with the number of fertile tillers·m−2, spikes per fertile tillers, and seed setting rate (Fig. 2d).
Figure 2.
Correlation between the reproductive traits of Dactylis glomerata. The correlation heat map visualization of (a) control, (b) CCC, (c) TE, and (d) CCC + TE are presented. ** significant correlation at the 0.01 level; * significant correlation at the level of 0.05.
Principal component analysis of the traits
-
Principal component analysis of the investigated traits showed that under control treatment (Fig. 3a), among the 15 factors examined, the eigenvalues greater than 1 were the first two principal components, and the cumulative contribution rate reached 100%, representing most of the original data information. The contribution rate of the first principal component was 76.1%. The width of the flag leaf and internode number were the main positive contributions whereas the internode length was the main negative contribution with a factor coefficient of −0.31. On the other hand, the contribution rate of the second principal component was 24.0%, while the length of the top second leaf and actual seed yield were the main positive contributions. The actual seed yield had a major positive contribution, with a factor coefficient as high as 0.52 (Supplementary Tables S1 & S2).
Figure 3.
Principal component analysis of morphological and reproductive traits of Dactylis glomerata under different PGRs of treatment, (a) CK, (b) CCC, (c) TE, and (d) CCC + TE. Arrows represent physiological and reproductive traits with various lengths based on the impact of each trait on the separation of treatments. The 15 traits parameters (PH Plant height, IN Internode number, IL Internode length, SD Stem diameter, FLW Flag leaf width, FLL Flag leaf length, WTSL Width of top second leaf, LTSL Length of top second leaf, NFT The number of fertile tillers, SFT Spikes per fertile tillers, FS Florets per spikelet, SSR Seed setting rate, TSW 1000-seed weight, ASY Actual seed yield, TSY Theoretical seed yield) are shown.
When treated with CCC (Fig. 3b), among the 15 factors examined, those with eigenvalues greater than 1 were the first three principal components, and the cumulative contribution rate reached 92.1%. The contribution rate of the first principal component was 62.8%. Most of the traits were positive contributions, except for the internode number, flag leaf width, top second leaf width, and 1000-grain weight. The internode length and the actual seed yield had a major positive contribution, both with a factor coefficient of 0.32. The contribution ratio of the second principal component was 19.6%. All the traits were positive contributions, excluding plant height, length of the top second leaf, and florets per spikelet. Stem diameter had a major positive contribution, with a factor coefficient of 0.50. The third main component contributed 9.7%, and the 1000-grain weight was the main positive contributor, with a factor coefficient of 0.68 (Supplementary Tables S3 & S4).
Under TE treatment (Fig. 3c), among the 15 factors examined, the eigenvalues greater than 1 were the first four principal components, and the cumulative contribution rate reached 94.0%. The contribution rate of the first principal component was 55.2%. All other traits were positive contributions, except for stem diameter, flag leaf width, top second leaf width, and florets per spikelet. The contribution rate of the second principal component was 18.9%, and the number of fertile tillers was the largest positive contributor to the component, with a factor coefficient of 0.48. The third main component contributes 13.5%, with the flag leaf width and the width of the top second leaf as the main positive contributions, both with a factor coefficient of 0.42. The fourth main component contribution rate was 6.4%, and the major positive contribution was the stem diameter, with a factor coefficient as high as 0.62 (Supplementary Tables S5 & S6).
Under CCC + TE treatment (Fig. 3d), among the 15 factors examined, the eigenvalues greater than 1 were the first three principal components, and the cumulative contribution rate occupied 91.41%. The contribution rate of the first principal component was 37.7%. All the traits were positive contributions, except for plant height, internode number, width of the top second leaf, and florets per spikelet. The number of fertile tillers had a major positive contribution, with a factor coefficient of 0.40. The contribution ratio of the second principal component was 33.7%, with the flag leaf width as the main positive contribution and factor coefficient of 0.43. The third main component contributed 19.0%, and the plant height was the main positive contribution, with a factor coefficient as high as 0.54 (Supplementary Tables S7 & S8).
Effects of PGRs at different concentrations on actual seed yield of Dactylis glomerata
-
In the two-year experiment, the actual seed yield was different under different treatments of orchardgrass. Low concentrations of CCC increased seed yield, with the highest yield increase rate at 17.3%, but when the concentration reached 1,250 g·hm−2, it reduced the yield of orchardgrass, which was decreased by 14.0%. TE increased seed yield at low concentrations but decreased seed yield at high concentrations. Within the range of tested concentrations, the mixture of CCC + TE increased the seed yield of orchardgrass by 47.9% when the concentration was 500 + 150 g·hm−2 (Table 5).
Table 5. Effect of PGRs of different concentrations on the actual seed yield of Dactylis glomerata in 2017−2018.
PGR No. Actual seed yield (kg·hm−2) Increase production compared with the control (kg·hm−2) Yield increase rate (%) CK 216.3 − − A A1 253.7 37.3 17.26 A2 248.4 32.1 14.85 A3 221.7 5.4 2.49 A4 186.1 −30.2 −13.97 B B1 278.3 62.0 28.65 B2 297.1 80.7 37.32 B3 240.8 24.5 11.32 B4 209.2 −7.1 −3.29 C C1 269.5 53.1 24.56 C2 320.0 103.7 47.91 C3 280.2 63.9 29.53 C4 255.7 39.4 18.22 Effects of PGRs at different concentrations on the microstructure of vascular bundle cells of Dactylis glomerata
-
The different concentrations of PGRs affected the seed yield and the vascular bundle tissue cells of orchardgrass stems. Under CCC treatment, the number of sclerenchyma cells increased with the increased concentration but reached the maximum when the concentration was 1,000 g·hm−2. The change of xylem cell number was consistent with that of sclerenchyma cells, reaching the maximum at 1,000 g·hm−2. The number of xylem cell layers was three except for the control under CCC treatment. The number of sclerenchyma cells was first increased and then decreased with the increase in TE concentrations, reached the maximum at 200 g·hm−2, and compared with the control, the number of sclerenchyma cells increased under all TE concentrations. Similarly, the changes in xylem cell number and layer number were consistent with the changes in sclerenchyma cell number, which first increased and then decreased with the increase in concentration. Generally, all treatments had two layers, except for concentrations at 100 and 200 g·hm−2, which had three layers. When CCC + TE were applied in combination at a concentration of 1,000 + 150 g·hm−2, the number of sclerenchyma cells was the highest, with 40 sclerenchyma cells. In addition, the number and layer of xylem cells first increased and then decreased with the increase in concentration. Among them, the mixed treatment of CCC + TE with concentration at 500 + 150 g·hm−2 had the best effect, which was significantly higher than other treatments. There was no significant change in cell layer numbers of sclerenchyma under all treatment concentrations (Table 6).
Table 6. Effect of PGRs of different concentrations on the vascular bundle cells of Dactylis glomerata.
PGR No. Sclerenchyma Xylem Cell
populationCellular
layerCell
populationCellular
layerA CK 27c 2a 8b 2b A1 32b 2a 13ab 3a A2 35a 2a 13ab 3a A3 36a 2a 14a 3a A4 35a 2a 14ab 3a B CK 27c 2a 8c 2b B1 38a 2a 12b 3a B2 38a 2a 14a 3a B3 36b 2a 14ab 2b B4 36b 2a 8c 2b C CK 27c 2a 8c 2c C1 26c 2a 11b 3b C2 37ab 2a 17a 4a C3 35b 2a 12b 3b C4 40a 2a 11b 3b Different letters in the same column indicate significant differences at the p < 0.05 level. The biopsy tissue showed that the stem vascular bundle of orchardgrass treated with CCC, TE, CCC + TE became larger than the untreated control (Fig. 4a−d). Sclerenchyma could thicken the cell walls. After being treated with CCC + TE, the xylem cells were not only more numerous but also larger (Fig. 4c). Based on the effects of these PGRs on vascular bundle cell populations in the stem of orchardgrass, and the effects of combined PGRs on various phenotypic traits of orchardgrass, the use of PGRs could increase the stem diameter, shorten the internode length and plant height, etc. by increasing the number of sclerenchyma cells and layers as well as the number of xylem cells and layers (Table 3, Fig. 4). Thus, the orchardgrass had better resistance to lodging during the harvest period and facilitated the transportation of assimilated substances into grains for storage. It might be due to the promotion of reproductive growth in orchardgrass at the expense of vegetative growth, thereby directly or indirectly increasing the seed yield.
Figure 4.
Transverse section of vascular bundle of Dactylis glomerata. The microstructure of vascular bundle cells of (a) CCC, (b) TE, (c) CCC + TE, and (d) control are shown. All the images are at 400 x microscopic. The cells between the two black circles were sclerenchyma cells as shown in (a) and the arrows point to phloem and xylem in (b).
-
Seed yield is the primary commercial feature for grass species[26]. The chlorocholine chloride (CCC) and trinexapac-ethyl (TE) used in this study are plant growth inhibitors, which could improve the seed yield of orchardgrass by regulating vegetative growth and promoting reproductive growth[27]. In this study, different plant growth regulators were applied at the GS32 period to investigate the effects on the agronomic traits and seed yield of orchardgrass. It was found that within a certain concentration range, all the treatments (CCC, TE, and CCC + TE) reduced the plant height of orchardgrass, while the stem diameter was significantly increased. Meanwhile, the application of CCC and TE might reduce lodging by lowering plant height, while mixed application of CCC + TE might reduce lodging by increasing stem diameter. The effects of CCC and TE on the reduction of plant height of orchardgrass might be different. The results show that CCC may reduce the plant height by reducing the internode length, while TE reduced the plant height by reducing the internode number, thereby, reducing potential lodging and increasing seed yield. Chastain et al. showed that TE reduced the stem length, and the higher the concentration, the greater the reduction. The increases in tall fescue seed yield attributable to TE were the result of increased seed number m−2[17]. Previous studies on the application of PGRs to maize (Zea mays)[28], wheat (Triticum aestivum)[29], and papaya (Chaenomeles sinensis)[30] reported that PGRs not only affected the seed yield but also affected the content of endogenous hormones in seeds, such as IAA, ABA, Z + ZR, GA, etc., and the activity of amylase. Although the content of relevant endogenous hormones and enzymes were not determined in this experiment, but the use of TE and CCC might have certain effects on the endogenous hormones and amylase of seeds, according to the results of previous studies.
Under different plant growth regulators, the relationship between the morphological and reproductive traits and the yield of orchardgrass was different. In the control treatment, plant height and yield were negatively correlated. In comparison, plant height and yield were positively correlated when treated with plant growth regulators. In alfalfa, spraying of CCC promoted reproductive development and increased seed yield by reducing plant height[31]. Besides that, the relationship between plant height and seed yield was different under different treatments. It might be based on the premise that plant growth regulators significantly reduced the plant height of orchardgrass. Reduced plant height might lead to high seed yield. Under this circumstance, lodging probably was no longer the main factor affecting seed yield. Chynoweth et al. found that reduced stem length was associated with increased perennial ryegrass seed yield[32]. In this experiment, there was a significant positive correlation between internode length and yield of orchardgrass under the treatment of CCC and TE, but there was no significant correlation between internode number and internode length and seed yield under the treatment of the mixture of CCC and TE. These results showed that internode number and internode length might not be the main factors affecting seed yield under the mixture of CCC and TE. Flag leaf and top second leaf are the important functional leaves of plants for seed production yield[33]. It has been reported that flag leaf size had a significant positive effect on the seed yield of Festuca pratensis[34]. In this experiment, under different plant growth regulators, the correlation between the width and length of the flag leaf and the top second leaf and actual seed yield were different. For instance, under the treatment of CCC and TE, the length of the top second leaf had a significant positive correlation with the actual seed yield, while under the mixed treatment of CCC + TE, had a negative correlation with the seed yield. This might be caused by the simultaneous use of CCC + TE which had some antagonistic effects on the traits of orchardgrass[35].
The decrease in seed yield was correlated with a decrease in reproductive tiller number, fewer spikelets per reproductive tiller, and fewer seeds per spikelet[36]. In a specific concentration range, different plant growth regulator treatments significantly increased the number of fertile tillers·m−2, spikes per fertile tillers, and seed setting rate. However, when the concentration exceeded a certain range, it would reduce the seed yield of orchardgrass, such as the concentration of CCC at 1,250 g·hm−2 and the concentration of TE at 400 g·hm−2, there was a decrease in seed yield. In this experiment, under different plant growth regulators, the number of fertile tillers·m2 was significantly positively correlated with the yield, and under the treatment of TE and the mixture of CCC + TE, the number of spikelets was also significantly positively correlated with the yield of orchardgrass. These results showed that different plant growth regulators might mainly increase the seed yield of orchardgrass by increasing the number of fertile tillers·m−2 and spikelets. The study by Tao et al. reported a similar results[37].
Zhang et al. showed that the seed yield of alfalfa could be increased by spraying CCC[31]. In an experiment conducted in Oregon (USA), the two-year experimental data showed that the yield of Trifolium pratense increased by 18.7% after spraying TE[38]. In addition, Rolston et al. proved that the application of TE could increase seed yield of perennial ryegrass seed crops[39]. TE is beneficial to increase the tiller number of perennial ryegrass[40]. In a certain concentration range, different plant growth regulator treatments significantly increased the number of fertile tillers·m−2, spikes per fertile tillers, and seed setting rate, but when the concentration exceeded a certain range, it would reduce the seed yield of orchardgrass, such as the seed yield decreased when the concentration of CCC was 1,250 g·hm−2 and the concentration of TE was 400 g·hm−2. PGRs effectually control lodging during pollination and set filling, resulting in better light interception. However, a high application rate combined with drought might leave seed crops standing upright and exposed to wind[9]. In the 2-year experiment, under high concentrations of CCC, the seed yield of orchardgrass decreased. In 2017, high concentrations of TE also reduced the seed yield. In western Oregon (USA), the application of paclobutrazol at low rates can improve seed yields in Festuca rubra and Festuca arundinacea, but not in orchardgrass[41]. However, in this experiment, the results showed that low doses of plant growth regulators increased the seed yield of orchardgrass. The conflicting results might be due to the difference in plant growth regulators used for both experiments. In the experiment by Young et al., although paclobutrazol did not affect fertile tiller numbers in other years, it increased the number of seeds per unit area in 1985[41]. During the production of gramineous plant seeds, lodging could reduce the seed yield during the growth stages of plants. However, the good effects of the sclerenchymal tissue thickness and the number of vascular bundles on plant lodging resistance according to a lot of studies[42,43], is positively correlated with plant lodging resistance[44]. In this study, the number of vascular bundles and sclerenchyma tissue thickness of orchardgrass increased after treatment with CCC, TE, and CCC + TE. Therefore, the application of PGRs might be indirectly beneficial to the lodging resistance in the seed production of orchardgrass. In addition, it might be due to the improvement of the vascular bundle cell populations, which in turn promotes the transportation of assimilating substances to the grain storage, to directly or indirectly improve the yield of orchardgrass seeds[45,46].
In terms of seed yield in two years, the yield-increasing effect of the mixture of CCC and TE was bigger than that of the single plant growth regulator. During the actual seed production in 2017−2018, when the mixture concentration of CCC and TE was 500 + 150 g·hm−2, the seed production reached its maximum, with an increase of 47.9% compared with the control. This is consistent with the results from previous studies which showed that the effect of mixed application of the same type of growth regulators on seed yield was bigger than that of single application of a regulator[47]. The comparison experiment of single and mixed application of chlormequat chloride and choline chloride was also carried out on winter wheat. The experiment proved that the mixed application further increased the winter wheat yield by about 8%[48]. In the mixed application of CCC + TE, the increase in seed yield of orchardgrass was mainly achieved by increasing the number of fertile tillers·m−2, florets per spikelet, and seed setting rate. Different plant growth regulators have significantly improved the theoretical seed yield and actual seed yield, but they are very different. The spring defoliation management of white clover seed crops had been shown to have a significant influence on the seed yield potential[49]. Procedures such as harvesting, handling, picking, and threshing during forage seed harvesting or even diseases will cause seed loss, thereby reducing actual seed yield. Therefore, spraying of the plant growth regulator in combination with good management practices can maximize the actual seed yield.
-
Plant growth regulators play an important role in forage seed production. 'Dianbei' Dactylis as a high-quality forage species, significantly increased its actual seed yield after spraying with certain concentrations of PGRs. Through the effects of different PGRs and different concentrations on the characteristics and seed yield of orchardgrass, it was found that the plant height of orchardgrass was significantly reduced by spraying two PGRs alone, and the stem diameter of Dactylis glomerata increased by spraying a mixture of CCC + TE. Different PGRs had different effects on the functional leaves (flag leaf and the top second leaf) of orchardgrass under different concentrations. Spraying two types of PGRs together in a certain range of concentrations could significantly improve the seed yield, and the microstructure of the stem, but when the concentration was too high, the seed yield was reduced. Among them, the mixture of CCC + TE at a concentration of 500 + 150 g·hm−2, resulted in the largest seed yield. Therefore, this PGR mixture is recommended to promote seed yield in orchardgrass production.
-
The authors confirm contribution to the paper as follows: study conception and design: Zhang R, Zhang X; data collection: Zhang R, Zhang X, Yang Z; analysis and interpretation of results: Zhang R, Rolston P, Yang Z, Feng G; draft manuscript preparation: Zhang R; supervision, resources, funding acquisition: Huang L, Zhang XQ, Nie G. All authors reviewed the results and approved the final version of the manuscript.
-
All data generated or analyzed during this study are included in this published article and its supplementary information files, and are available from the corresponding author upon reasonable request.
This research work was supported by the earmarked fund for CARS (CARS-34), and the Sichuan Province Breeding Research grant (Grant No. 2021YFYZ0013).
-
The authors declare that they have no conflict of interest.
- Supplementary Table S1 Eigenvalue, contribution rate and cumulative contribution rate of main components of traits under CK.
- Supplementary Table S2 Component matrix among traits under CK.
- Supplementary Table S3 Eigenvalue, contribution rate and cumulative contribution rate of main components of traits under CCC.
- Supplementary Table S4 Component matrix among traits under CCC.
- Supplementary Table S5 Eigenvalue, contribution rate and cumulative contribution rate of main components of traits under TE.
- Supplementary Table S6 Component matrix among traits under TE.
- Supplementary Table S7 Eigenvalue, contribution rate and cumulative contribution rate of main components of traits under CCC+TE.
- Supplementary Table S8 Component matrix among traits under CCC+TE.
- Copyright: © 2024 by the author(s). Published by Maximum Academic Press, Fayetteville, GA. This article is an open access article distributed under Creative Commons Attribution License (CC BY 4.0), visit https://creativecommons.org/licenses/by/4.0/.
-
About this article
Cite this article
Zhang R, Zhang X, Rolston P, Yang Z, Feng G, et al. 2024. Effects of different plant growth regulators on phenotypic variation and seed yield of Dactylis glomerata. Grass Research 4: e022 doi: 10.48130/grares-0024-0021
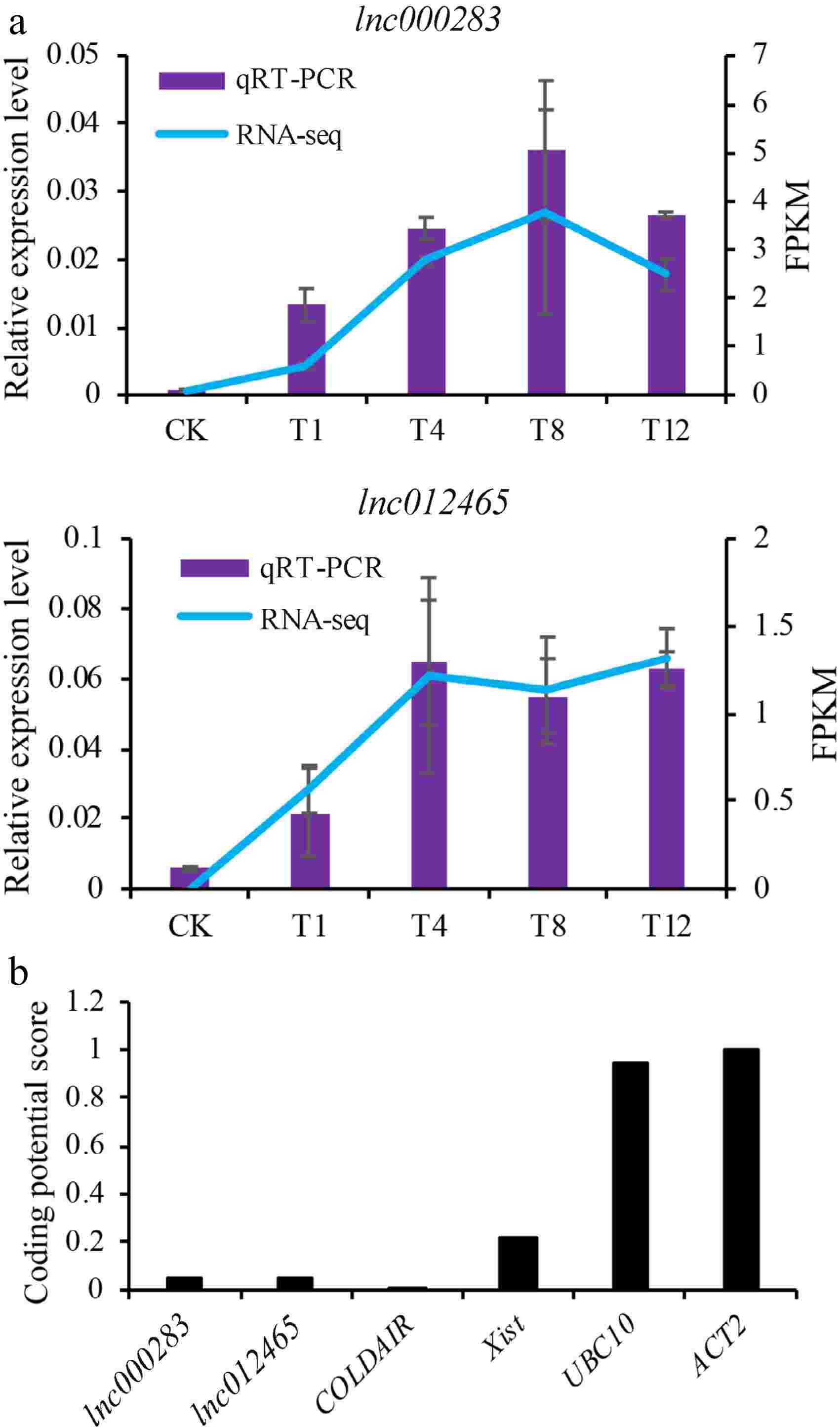