-
Cassava (Manihot esculenta Crantz, 2n = 36) is a perennial species belonging to the Manihot genus in the Euphorbiaceae family. It is the sixth most important food crop, and its cultivation supports the livelihoods of over 800 million people in tropical regions, providing a vital source of carbohydrates amidst global food scarcity[1]. In addition to its primary role as a food crop, cassava's storage roots are extensively utilized in industrial processes due to their high starch content.
Cassava typically contains starch levels ranging from 25% to 35% in fresh weight, and over 85% of its dry weight. The starch exhibits distinctive physical and chemical properties, including high viscosity, transparency, and freeze-thaw stability. Both natural and modified cassava starch have demonstrated notable health benefits, such as reducing cholesterol, regulating blood sugar, and offering anti-diabetic properties, making it a key focus in functional food development. Additionally, yellow- or purple-fleshed cassava is rich in carotenes and other flavonoids, enhancing its value as a biofortified food source. This attribute positions cassava as an effective means of addressing nutritional deficiencies, particularly in regions with limited food availability.
Cassava exhibits characteristics of both C3 and C4 photosynthesis, demonstrating a high photosynthetic rate and minimal light respiration under suitable conditions, which allows for efficient conversion of solar energy into carbohydrates per unit area[2−4]. Its resilience is further highlighted by its drought tolerance, enabling growth in semi-arid and arid regions, where it can withstand both short-term and prolonged droughts of 4−6 months[5]. Additionally, cassava demonstrates rapid recovery from drought stress, owing to specific physiological traits such as its stem and leaf morphology, stomatal regulation, and extensive root system. Cassava also efficiently absorbs nutrients from low-nutrient soils, allowing it to thrive even in infertile conditions.
However, cassava productivity faces significant challenges due to the spread of cassava mosaic disease (CMD) and cassava brown streak virus disease (CBSD), two prevalent viral diseases affecting large areas of Africa[6,7]. CMD, caused by various species of begomoviruses is characterized by distinctive mosaic patterns on leaves, stunted growth, and reduced root yield. If left unchecked, CMD can result in complete crop failure, posing a severe threat to food security in affected regions[8,9]. Similarly, CBSD, caused by viruses from the Potyviridae family, produces brown streaks on storage roots, leading to rot and rendering the roots inedible. Both diseases not only reduce yield but also compromise root quality, thereby diminishing the overall nutritional value of cassava. Developing resistant cultivars and implementing effective disease management strategies are crucial for mitigating the adverse impacts of CMD and CBSD on cassava productivity and ensuring food security in vulnerable regions. Although reliable sources of resistance to CMD and CBSD have been identified[10,11], the introgression of these resistance traits using conventional backcross breeding is not feasible in cassava[12].
There is a pressing imperative to enhance various agronomic traits of cassava. For instance, cassava storage roots contain low levels of proteins (< 1% in dry weight) and other essential nutrients, necessitating biofortification efforts to improve its nutritional profile. Additionally, cassava plants contain cyanogenic glycosides, which, if improperly processed, can generate toxic hydrogen cyanide, posing significant health risks to humans[13]. Cassava's susceptibility to cold stress and post-harvest physiological deterioration (PPD) further limits its cultivation and utility outside tropical and subtropical regions[14−16]. Moreover, the branching patterns of cassava significantly affect the quality of seed stems and present challenges for mechanized management[17]. Weed competition is another significant biological stress that impacts cassava production[18]. These challenges collectively hinder the advancement and broader utilization of the cassava industry.
In recent decades, a more dynamic recurrent selection system has been implemented to improve cassava varieties. However, traditional breeding approaches are often hindered by challenges such as delayed flowering, limited flower production, self-incompatibility, low seed yield, and the crop's inherent high heterozygosity, making these methods both time-consuming, and labor-intensive[2,19]. With advancements in the cassava reference genome and genome assemblies of various accessions[20,21], biotechnology tools have been adapted for cassava improvement. Molecular mapping and marker-assisted selection are now employed for key traits selection and genetic transformation has been utilized to transfer beneficial genes, significantly enhancing cassava quality[22]. Particularly genome editing, have emerged as efficient tools for introducing desired traits into cassava breeding programs over the past five years.
-
Genome editing is a powerful tool for making precise genetic modifications in crops, essential for meeting the demands of a growing population. Techniques such as zinc finger nucleases (ZFNs), transcription activator-like effector nucleases (TALENs), and CRISPR/Cas have proven effective in plant genome editing. Unlike ZFNs and TALENs, CRISPR-Cas9 relies on single guide RNA (sgRNA) for simplicity and high efficiency, eliminating the need for extensive protein engineering[23]. Derived from bacterial defense mechanisms, the CRISPR/Cas system, particularly the type II system from Streptococcus pyogenes, is widely employed for genome editing[24,25]. This system involves a complex of trans-activating RNA (tracrRNA) and Cas protein that guides the Cas9 endonuclease to target specific genomic sequences. Engineered sgRNAs streamline CRISPR/Cas9 manipulation by directing Cas9 to induce double-stranded DNA cleavage near the protospacer adjacent motif (PAM)[26]. Repair mechanisms such as non-homologous end joining (NHEJ) introduce mutations for gene knockout (KO)[27], while homologous-directed repair (HDR) facilitates gene substitution or knock-in (KI)[28].
Cells typically employ the NHEJ repair pathway, resulting in random individual base deletions or insertions (Indels) at target sites[29]. While HDR offers high fidelity, its efficiency is often low, with only a few endogenous plant genes accurately repaired in previous studies[30]. Double-strand breaks (DSBs) induced by CRISPR/Cas9 can lead to genomic instability and reduced efficiency. To address this, precise genome editing methods using CRISPR/Cas9 have been developed to create base substitutions. Base editors, guided by sgRNA, utilize cytidine deaminase or a hypothetical DNA adenosine deaminase fused with Cas9n (D10A) to catalyze base transitions (C to T, G to A, A to G, and T to C) without inducing double-stranded DNA cleavage[31]. Additionally, tools like cytosine transversion base editors (CGBE), saturated targeted endogenous mutagenesis editors (STEME), and APOBEC-Cas9 fusion-induced deletion systems (AFID) enable precise base transitions, cytosine transversions, and multinucleotide deletions[32,33]. However, these editors may not cover all base conversions or precise insertions.
Prime editing, comprising Cas9n (H840A), a reverse transcriptase, and an engineered prime editing guide RNA (pegRNA), offers a solution by enabling any base conversion and insertions of target genes[34]. Both base editing and prime editing have demonstrated potential for correcting mutations, enabling gene knockout without DSB generation, and generating gain-of-function alleles such as herbicide tolerance and resistance to Magnaporthe oryzae in rice[35]. Undoubtedly, the rapid advancement of genome editing tools holds immense promise for crop breeding, facilitating the creation of novel varieties with desirable traits.
The CRISPR/Cas9 system enables the construction of large knockout mutant libraries for forward genetic screens, allowing the simultaneous targeting of multiple genes. Furthermore, it facilitates the identification of causal genes and addresses challenges such as genetic redundancy[36]. Multiplex genome editing, which involves targeting multiple DNA loci within a genome simultaneously is a powerful strategy for creating desirable genotypes and traits within a single generation[37].
-
Efficiency and specificity in plant genome editing are influenced by various factors, including Cas9 variants, Cas9 modifications, sgRNA sequence composition, target sites, and delivery methods. In addition to the widely used SpCas9, variants such as StCas9 from Streptococcus thermophilus[38] and the smaller SaCas9 from Staphylococcus aureus[39] have demonstrated functionality in plants, enhancing specificity through expanded PAM recognition[40]. The recently developed Cpf1 exhibits proficiency in generating DSBs with sticky ends, resulting in larger indels with increased specificity[41]. Cas9 can be modified to create catalytically inactive forms (dCas9) by mutating specific residues. This mutant retains DNA-binding activity and can be a repressor, hindering RNA polymerase binding to promoters or impeding its movement. Alternatively, dCas9 can be fused with gene activation or repression domains to facilitate CRISPR activation (CRISPRa) or CRISPR interference (CRISPRi). Activation domains include VP64, P65AD, VPR, Rta, and TEH1, while repression domains encompass KRAB, DNMTs, and LSD1. Complexes formed by sgRNA and dCas9 bind to target sites, regulating gene expression without inducing double-strand DNA cleavage.
The composition of sgRNA is pivotal in gene editing efficiency. Higher guanine-cytosine (GC) content in sgRNA has been shown to enhance editing efficiency[42]. Additionally, the length of sgRNA also influences target accuracy; typically, a length of 17 base pairs (bp) demonstrates higher editing efficiency compared to sgRNAs of 18−20 bp[43]. Moreover, the CRISPR/Cas9 system facilitates efficient intron-mediated site-specific gene replacement and insertion by employing a pair of sgRNAs targeting adjacent introns, alongside a donor DNA template with matching sgRNA sites[44].
Cas9 and sgRNA expression cassettes can be introduced into plant cells using various methods, including Agrobacterium-mediated transformation, particle bombardment, polyethylene glycol (PEG), viral vectors, and nanoparticles. Additionally, synthesized sgRNA can be delivered into plant cells that have already been transformed with Cas9 to induce target gene mutation[45]. However, Agrobacterium-mediated transformation raises regulatory concerns due to genome integration. To generate transgene-free mutations with CRISPR/Cas9, purified Cas9-sgRNA complexes, known as ribonucleoproteins (RNPs), transient expression of CRISPR/Cas9 DNA (TECCDNA) or transient expression of CRISPR/Cas9 RNA (TECCRNA) can be introduced into plant protoplasts[46−48]. While this approach reduces off-target mutations compared to DNA-based editing, it generally exhibits lower efficiency.
Moreover, DNA replicons derived from deconstructed geminiviruses have been employed to enhance CRISPR/Cas9 reagent delivery and DNA repair template, thereby improving gene targeting frequencies[49,50]. Ongoing advancements in CRISPR-based genome editing across both medical and agricultural fields signify significant growth in industrial biotechnology, yet its full potential remains largely untapped. In plant biology, which is characterized by quantitative traits and widespread polyploidy among vascular plants, multiplex gene editing holds promise for improving complex traits[51]. Furthermore, novel applications such as RNA cleavage[52] and chromatin imaging[53] may emerge from CRISPR/Cas9-derived tools. Emerging Cas9 mutants like SpG and SpRY enable nucleotide mutations beyond the proximal PAM sequence[54], further expanding genome editing possibilities. Continued technological advancements are anticipated, offering additional opportunities for agriculture to enhance disease resistance and yield indices.
-
The CRISPR/Cas9 system is renowned for its exceptional efficiency and cost-effectiveness, representing a significant advancement in genetic engineering (Fig. 1). Since its introduction, this groundbreaking technology has been rapidly adopted in cassava breeding, leading to a series of remarkable developments. It has been pivotal in prolonging the shelf-life of cassava storage roots[55] and strengthening disease resistance[56−60], as well as in conferring herbicide tolerance[61]. The applications of CRISPR/Cas9 in cassava improvement are diverse and far-reaching, extending to starch modification[62−64] and the reduction of cyanogenic glycoside content[65−67]. The system's versatility and effectiveness make it a critical tool in the ongoing efforts toward agricultural sustainability and global food security.
Figure 1.
Schematic flow of genome editing in cassava. An sgRNA is designed to target the exon or promoter region of specific genes, guided by the target sequence within the sgRNA. This sgRNA associates with Cas9 endonuclease or nickase, forming a Cas9-gRNA complex to enable nuclease editing, base editing or prime editing. The CRISPR/Cas9 binary construct can be delivered directly into cassava FECs through Agrobacterium or particle bombardment, or into protoplasts via PEG-mediated transfection, leading to genome modification. Alternatively, CRISPR/Cas9 components -- whether in the form of DNA (TECCDNA), RNA (TECCRNA) or pre-assembled ribonucleoproteins (RNPs) – are delivered into FECs via particle bombardment or into protoplasts via PEG, respectively. Once transformed, the regenerated protoplasts or FECs develop into plant seedlings under antibiotic selection. These seedlings are then screened for targeted mutations using DNA sequencing techniques such as PacBio SMRT sequencing, Hi-TOM, or Sanger sequencing. Furthermore, cassava plants can be transiently transformed via Agrobacterium tumefaciens infiltration, carrying viral vectors with the Cas9-sgRNA construct to induce gene knockouts. All identified mutants are subsequently evaluated in the field trials based on phenotypic traits and molecular analysis for breeding purposes.
The phytoene desaturase (PDS) gene is integral to the carotenoid biosynthesis pathway, with its loss resulting in dwarfism and albino phenotypes due to disruptions in chlorophyll, carotenoid, and gibberellin biosynthesis[68]. Given its critical role and observable phenotypic effects, PDS was selected as a primary marker to evaluate the efficacy of CRISPR/Cas9 in cassava genome editing[69]. Two gRNAs were specifically designed to target exon 13 of the MePDS gene in TME 204 and TMS60444 cultivars. Using Agrobacterium-mediated delivery of CRISPR/Cas9 reagents into cassava cells, 90%−100% albino phenotypes were produced in transformed cassava plants. Sequencing results confirmed mutations in all 38 plants, demonstrating the high efficiency of CRISPR/Cas9 in tropical cassava and underscoring its potential for broader agricultural applications[69].
Modifying starch quality
-
Cassava storage roots are highly valued for their abundant starch content, which is crucial for both carbohydrate supply and various industrial applications. Native cassava starch predominantly comprises amylose (20%−30%) and amylopectin (70%−80%). Despite being present in smaller amounts, amylose plays a significant role in determining starch properties, especially in terms of pasting and gelatinization behavior. On the other hand, amylopectin, with its complex branching structure, primarily forms the granule matrix and contributes to the starch's crystallinity[70]. Starch biosynthesis in cassava is governed by several key enzymes, including granule-bound starch synthase (GBSS) and protein targeting to starch (PTST1), which are critical for amylose synthesis[71]. Additionally, soluble starch synthase (SS), starch branching enzyme (BE), and debranching enzyme (DBE), play vital roles in amylopectin formation. The intricate balance between amylose content and the structure of amylopectin significantly influences the overall characteristics of the starch[72].
Recent advancements in gene editing techniques, particularly CRISPR/Cas9, have enabled precise manipulation of starch biosynthetic pathways in cassava, as evidenced by previous studies[62−64]. Through the integration of the Arabidopsis FLOWERING LOCUS T gene, mutations in GBSSI and PTST1 have produced cassava varieties with altered gelatinization properties in starch, while also confirming the transgene-free inheritance of these traits[62]. Additionally, CRISPR/Cas9-mediated editing of MeSSIII and SBE2 genes has led to the creation of mutants. While no gene-edited germplasm has been released, these mutants are invaluable for fundamental research and for advancing our understanding of starch biosynthesis. This has resulted in the development of high-amylose cassava varieties with enhanced industrial and nutritional potential[62−64]. Collectively, these scientific endeavors underscore the effectiveness of CRISPR/Cas9-mediated mutagenesis in manipulating starch biosynthetic genes in cassava, affirming CRISPR/Cas9 as a powerful tool for producing cassava starch with tailored characteristics for diverse applications.
Detoxifying cyanides
-
Cassava contains cyanogenic glycosides, primarily linamarin and lotaustralin, across all tissues except seeds. Hydrolysis of these glycosides releases hydrogen cyanide (HCN), which is toxic to parasites, herbivores, and humans[73,74]. While cyanogenic glycosides function as natural herbivore repellents and play a role in nitrogen transport[75], inadequate processing of cassava can lead to cyanide-related health risks. Conventional processing methods, such as fermentation and cooking, are labor-intensive and may not fully eliminate cyanogens, potentially resulting in harmful food products[76,77].
To address this, two primary strategies have emerged: the development of cyanogen-free cassava plants and the creation of transgenic varieties to enhance cyanide turnover during processing[78]. The first step in cyanogen biosynthesis involves enzymes encoded by paralogous genes CYP79D1 and CYP79D2[79]. Targeting CYP79D1 via CRISPR/Cas9-mediated mutagenesis has led to a significant reduction in cyanogenic glycosides[65]. Furthermore, the simultaneous targeting of both CYP79D1 and CYP79D2 have successfully eliminated cyanogenic potential in various cassava cultivars[66]. However, due to the high sequence similarity between these genes, targeting CYP79D1 might inadvertently affect CYP79D2, contributing to the reduction of cyanogenic glycosides[65]. Additionally, knocking out the cyanogenic glucoside transporter MeCGTR1 via CRISPR/Cas9 has resulted in lower cyanogenic glycoside levels in leaves and stems[80]. Furthermore, α-hydroxynitrile lyase gene MeHNL catalyzing the decomposition of cyanogenic glycosides were edited using CRISPR/Cas9 technology, 26 mutant plants were verified and hydrocyanic acid and cyanogenic glycosides in the mutant lines were significantly reduced[67]. These CRISPR/Cas9-based approaches not only enhance our understanding of cyanogen roles in cassava but also demonstrate the feasibility of generating acyanogenic cassava cultivars.
Increasing herbicide resistance
-
Weeds pose a significant challenge to cassava production by competing for vital nutrients, which hinders cassava growth. Effective weed control is essential for optimizing cassava yields, with herbicides being a commonly employed solution. Enhancing the herbicide tolerance of cassava cultivars has, therefore, become a critical objective.
Glyphosate, a widely used herbicide, is particularly effective in weed management. To bolster glyphosate tolerance in cassava, researchers have focused on the native and constitutive promoter regions of the EPSPS (5-enolpyruvylshikimate-3-phosphate synthase) gene, in addition to exploring various paired amino acid substitutions. Using CRISPR/Cas9-mediated gene editing techniques, scientists have aimed to introduce simultaneous modifications, including both the EPSPS promoter swap and dual amino acid substitutions. These efforts have led to the development of EPSPS-edited cassava plants that exhibit remarkable resilience to glyphosate, as demonstrated in studies such as that conducted by Hummel et al.[61]. This breakthrough holds significant promise for fortifying cassava crops against weed pressures, thereby optimizing productivity in agricultural settings.
Improving bacterial disease resistance
-
Cassava, is a vital staple food, and the world's fourth-largest source of calories faces significant challenges from bacterial, viral, and pest diseases that severely impact its production and yield. Among these threats, cassava bacterial blight (CBB), caused by Xanthomonas axonopodis pv. manihotis (Xam), is particularly devastating and affects regions where cassava is cultivated[81]. The Xam668 strain, known for its high virulence, represents a formidable threat by deploying the major virulence determinant TALE20 Xam668 effector into cassava cells[82,83]. This effector, equipped with repeat variable diresidues (RVDs), targets the effector-binding element (EBE) on the MeSWEET10a promoter. Activation of this promoter leads to the expression of the MeSWEET10a gene, which encodes a protein responsible for transporting sucrose and other sugars from the interior of plant cells to the apoplast. This process provides carbon sources that support pathogen bacteria reproduction[82,83].
In response to the CBB threat, several strategies have been developed to mitigate CBB symptoms effectively. One approach involves inhibiting the expression of the MeSWEET10a gene, which has been shown to reduce CBB symptoms significantly[58]. Using CRISPR-mediated HDR, researchers inserted a GFP gene at the 3' end of MeSWEET10a, allowing real-time visualization of the initial stages of CBB infection in vivo[58]. Additionally, the important role of the EBETALE20 region in the MeSWEET10a promoter for regulating MeSWEET10a gene expression was confirmed through dual luciferase reporter assays[59]. CRISPR/Cas9-mediated gene editing of EBETALE20 in the MeSWEET10a promoter in the SC8 cultivar resulted in mutants with enhanced resistance to Xam11, without significant differences in key yield-related traits compared to wild-type plants[59]. Another innovative approach involved epigenome editing. A synthetic zinc-finger DNA binding domain, fused with an RNA-mediated DNA methylation component, was designed to methylate the TAL20 EBE of the MeSWEET10a promoter. This methylation blocked TALE20 binding, leading to MeSWEET10a inactivation and reduced CBB symptoms[60].
This epigenetic editing approach offers several advantages, including smaller transgenes and flexible PAM site locations. Its stability in inheritance and potential applicability to other crops highlight promising avenues for further research and implementation[60].
The higher conserved sequences of TALE in promoter or ribosome-binding site (RBS) were knockdown through CRISPRi strategy and CBB symptoms could be significantly reduced, and this system was also used to search for candidate cassava genes targeted by Xpm[84].
Editing of the MeSWEET10a TAL20 EBE and/or coding sequence by Elliott et al.[85] has demonstrated a reduction in CBB disease symptoms following Xpm infection in mutant lines. Viable F1 progeny with intact coding sequence but promoter mutations further validated that blocking MeSWEET10a induction is an effective strategy to reduce CBB symptoms. This approach underscores the importance of inducing promoter mutations that obstruct the TAL effector binding site[85].
Sclerotium rolfsii presents a severe threat to cassava plants, affecting both young and mature cassava plants and causing yield losses of up to 80%[86,87]. To combat this challenge, a novel CRISPR/Cas9 technology has been developed for the detection of Sclerotium rolfsii. This advanced method integrates recombinase polymerase amplification (RPA) with CRISPR/Cas12a, offering superior performance compared to traditional PCR detection methods. This innovative approach promises enhanced surveillance of pathogen infections, as demonstrated in a previous studies by Changtor et al.[88].
Improving viral disease resistance
-
CBSD poses a serious threat to both cassava food security and human consumption. This disease is attributed to Cassava brown streak virus (CBSV) and Ugandan cassava brown streak virus (UCBSV), which are members of the Potyviridae family (Genus: Ipomovirus). These viruses exploit eukaryotic translation initiation factor 4E (eIF4E) or its analogs to initiate the translation of viral genomic RNA within host cells. Notably, the eIF4E family comprises five transcripts: one eIF4E, two eIF(iso)4E, and two novel cap-binding proteins (nCBPs)[89]. Among these, nCBP1 and nCBP-2 are integral components of eIF4E and play a pivotal role in interacting with the viral genome-linked protein (VPg). By employing CRISPR/Cas9 to target both nCBP-1 and nCBP-2, researchers have significantly reduced CBSD symptoms and CBSV accumulation in storage roots. This approach also lessens the severity and incidence of storage root necrosis[56].
In a recent study, CRISPR/Cas9 technology was used to confer resistance to African cassava mosaic virus (ACMV) geminiviruses in cassava plants. Despite initial optimism, the transgenic plants did not exhibit enhanced geminivirus resistance due to the emergence of a conserved mutant virus, leading to viral escapes. This highlights the imperative for caution when using CRISPR/Cas9 to enhance virus resistance, as it may inadvertently result in the evolution of resistant viral strains[57]. Future investigations should address factors such as the optimal ratio of Cas9 to sgRNA, the specific Cas9 variant used, and the efficacy of stronger promoters in enhancing cassava virus resistance through CRISPR/Cas9 approaches[90].
South African cassava mosaic virus (SACMV), a member of the cassava mosaic geminivirus species transmitted by whiteflies, is responsible for CMD[91]. CMD outbreaks significantly impact cassava yields and food security[8,9]. To elucidate host responses to SACMV infection, protoplasts derived from both SACMV-susceptible and tolerant cassava genotypes were transformed with SACMV infectious clones and subjected to CRISPR/Cas9-mediated editing targeting (ubiquitin RING E3 ligase) MeE3L[92]. This approach enabled high-throughput screening of genes involved in SACMV response pathways and confirmed the pivotal role of cassava E3 ligase in these pathways[92]. Additionally, protoplasts isolated from the SACMV-tolerant cassava cultivar TME3 was transfected to knock down the expression of the cassava coiled-coil nucleotide-binding leucine-rich repeat (CC-NLR) protein MeRPPL1 using CRISPR/Cas9. This demonstrated that MeRPPL1 contributes to geminivirus tolerance in TME3[93].
Prolonging shelf-life of cassava storage roots
-
PPD presents a significant challenge to cassava cultivation by reducing the shelf-life of storage roots due to oxidative stress and the accumulation of secondary metabolites during harvest. To address this issue, researchers have utilized CRISPR/Cas9-mediated mutagenesis techniques. The focus was initially on knocking out key enzymes involved in scopoletin biosynthesis, specifically the feruloyl CoA 6′-hydroxylase genes (MeF6'H1-MeF6'H2, MeF6'H1, and MeF6'H3). The resulting mutant cassava plants demonstrated an enhanced shelf-life and a notable reduction in scopoletin contents[55].
These findings highlight the effectiveness of the CRISPR/Cas9 system in cassava breeding efforts aimed at improving yield and quality (Table 1). By precisely targeting specific genetic elements involved in post-harvest attributes, CRISPR/Cas9 facilitates the development of cassava varieties with extended storage characteristics and reduced susceptibility to physiological deterioration.
Table 1. The applications of gene editing technology in cassava.
Gene names Gene functions Vectors Editing methods Mutant features Ref. MeSWEET10a Susceptibility (S) gene for CBB CRISPR-TA-coupled HDR strategy for tagging MeSWEET10a Target the 3' end of MeSWEET10a with HDR repair Susceptible to CBB infection (because GFP insertion is hemizygous) Veley et al.[58] MeSWEET10a Susceptibility (S) gene for CBB pCAMBIA1301-Cas9-EBE-sgRNA Target the EBETALE20 of MeSWEET10a promoter Improved resistance to CBB Wang et al.[59] MeSWEET10a Susceptibility (S) gene for CBB pEG302-DMS3 (DEFECTIVE IN MERISTEM SILENCING3)-ZF (artificial zinc-fingers)-EBE DNA methylation the EBETALE20 of MeSWEET10a promoter Improved resistance to CBB Veley et al.[60] MeSWEET10a Susceptibility (S) gene for CBB pUC18-mini-Tn7T-Gm-dCas9-sgRNA Target the higher conserved sequence of MeSWEET10a in promoters or 5' -UTR regions (ribosome binding site) Improved resistance to CBB Zárate-Chaves et al.[84] MeSWEET10a Susceptibility (S) gene for CBB pTRANS_220D-35S-Cas9-multiple gRNA spacer Csy4 array (gRNA1/2, gRNA1/3, gRNA4/5) Target the EBETALE20 of MeSWEET10a promoter and/or coding sequence Improved resistance to CBB Elliott et al.[85] nCBP1-nCBP2 Transcripts of eIF4E family comprises to induce CBSD pCAMBIA2300-Cas9-AtU6-26-gRNA (nCBP-1, nCBP-2, nCBP-1/nCBP-2) Target the exons of translation initiation factor 4E (eIF4E) isoforms nCBP-1, nCBP-2, nCBP-1 and nCBP-2 Double mutant lines delayed and attenuated CBSD aerial symptoms,
but single mutant lines notGomez et al.[56] MePDS Key enzymes in the carotenoid biosynthesis pCAMBIA2300-35S-Cas9-AtU6-26-gMePDS-1, gMePDS-2 Target two target sites in the exon 13 of MePDS Albino or partial albino phenotypes Odipio et al.[69] MeEPSPS Catalysing synthesis of aromatic amino acids and secondary metabolites in plant chloroplasts pCAMBIA2300-2x35S-Cas9-AtU6-sgMeEPSPS#7-At7SL-sgMeEPSPS#11 Target sites of second intron and promoter of MeEPSPS Achieving glyphosate tolerance Hummel et al.[61] MeGBSS, MePTST1 Synthesizing long-chain glucan amylose and CBM48-containing protein mediate GBSS localization to starch granules 35S-pcoCas9-eGFP-NLS-tHSP-psynU6-gMeGBSS/gMePTST1-AtFT Target the exon 2 of MeGBSS and an exon at the 3′ end of the coding sequence of MePTST1 Diminish or delete amylose Bull et al.[62] MeSSIII-1,
MeSSIII-2Synthesis of long chain in plant amylopectin glucan pCAMBIA1301-Cas9-AtU6-gMeSSIII-1 and gMeSSIII-2 Target the exons of MeSSIII-1 and MeSSIII-2 Resistant calli stage Li et al.[63] MeSBE2 Synthesis of short chain during amylopectin biosynthesis pCAMBIA1301-Cas9-AtU6-gMeSBE2 Target the second and fifth exons of MeSBE2 Increase amylose and resistant starch Luo et al.[64] MeCYP79D1 Cyanogen biosynthesis 35S-Cas9-AtU6-26-sgMeCYP79D1 Target the exon 3 of MeCYP79D1 Reduce the levels of linamarin and evolved cyanide Juma et al.[65] MeCYP79D1, MeCYP79D2 Cyanogen biosynthesis pCAMBIA2300-Cas9-AtU6-sgMeCYP79D1/sgMeCYP79D2/gMeCYP79D1-MeCYP79D2 Target the exons of MeCYP79D1 and MeCYP79D2, respectively, the exons of MeCYP79D1 and MeCYP79D2 simultaneously Reduce the biosynthesis of cyanide with both genes or MeCYP79D2 mutagenesis, but MeCYP79D1 not Gomez et al.[66] MeCGTR1 High-affinity transporter of cyanogenic gulcosides 2x35S-Cas9-AtU6-26-sgMeCGTR1 Target the exon 1 of MeCGTR1 Lower level of cyanogenic glucosides in the top leaves and stems, no difference in roots and bottom leaves Lieberman et al.[80] MeF6'H1, MeF6'H1- MeF6'H2, MeF6'H3 Conversion of 6′ hydroxyl feruloyl-CoA to scopoletin 35S-Cas9-AtU6-sgMeF6'H1/sgMeF6'H1-MeF6'H2/ /sgMeF6'H3 Target the exon 1 of MeF6'H1, exon 2 of MeF6'H3, and the exons of MeF6'H1 and MeF6'H2 concurrently Delay PPD Mukami et al.[55] AC2, AC3 AC2 coding for the multifunctional TrAP protein involved in gene activation, virus pathogenicity, and suppression of gene silencing, and the AC3 gene coding for the REn protein involved in replication enhancement 35S-Cas9-U6-sgAC2-AC3 Target the viral AC2 and AC3 No significant differences in disease incidence, symptom severity, or virus titres Mehta et al.[57] MeE3L Hijacking and redirection of ubiquitination by geminiviruses, associated with CMD2 resistance locus pC1380-TMV-Cas9-eGFP-U6-26-gMeE3L-1-gMeE3L-2 Target the exon of MeE3L SACMV DNA accumulation Chatukuta & Rey[92] MeRPPL1 Involved in CMD tolerance, recognize pathogen effectors and trigger plant effector-triggered immunity (ETI) pC1380-TMV-Cas9-eGFP-U6-26-gMeRPPL1-1- gMeRPPL1-2 Target the exon of MeRPPL1 SACMV-DNA A accumulation Ramulifho et al.[93] -
The successful implementation of CRISPR/Cas9-mediated genome editing in cassava heavily relies on effective transformation techniques[94]. Various approaches, such as using cotyledons from somatic embryos, friable embryogenic calli (FEC), and protoplasts derived from leaves and FEC, have been employed for cassava genetic transformation[95−97]. Among these methods, cotyledon transformation often results in cassava chimeras, while protoplast regeneration remains challenging due to low efficiency. Conversely, FEC and the embryogenic suspension cultures are more conducive to Agrobacterium-mediated transformation and particle bombardment. Particle bombardment offers several advantages, including the ability to bypass biological constraints, enabling the transformation of a wide range of plant species, and facilitating DNA-free gene editing[98,99] through the direct delivery of proteins, RNAs, and RNPs[100]. However, its application is limited by lower transformation efficiency. In contrast, Agrobacterium-mediated genetic transformation offers higher efficiency, reproducibility, simple equipment requirements, and stable transgene expression with single-copy integration.
Virus-induced gene silencing (VIGS) has also emerged as an alternative technique for introducing foreign genes and analyzing gene functions. This method has been used to confer resistance to cassava bacterial blight and to modify starch and dry matter content in storage roots[101−105]. Additionally, virus-induced genome editing (VIGE), using cassava common mosaic virus, has been successfully developed for cassava[106]. However, the limited cargo capacity of viral vectors necessitates the future use of smaller Cas effector proteins, replacing the larger Cas9[107].
Despite its utility, FEC induction remains highly genotype-dependent. The lengthy FEC induction process, coupled with subculturing, somatic embryogenesis, and plant regeneration, results in low transgenic plant production efficiency and high rates of somaclonal variation[108]. Establishing standard protocols for FEC-based transformation, including routine FEC induction and somatic embryogenesis for plant regeneration, is critical. Historically, the model cultivar TMS60444 has been widely used for genetic transformaton despite its lack of resistance to CMD or CBSD. In recent decades, significant efforts have improved FEC induction across various cultivars, including TME 204, TME 419, TME 3, TME 7, TME 14, TMS 01/0040, TMS 01/1206, TMS 91/02324, TMS 92/0326, KU50, and SC8[109−111]. Furthermore, methods to enhance cotyledonary embryo regeneration have been developed, such as gradually increasing BAP concentrations, using complete explants, adding silver nitrate (AgNO3), shortening subculture intervals[112] incorporating activated charcoal into maturation media, and applying desiccation treatments[113]. Flow cytometry is also used to analyze FEC ploidy, reducing the risk of regenerating of abnormal plants[114].
Novel approaches to improve genetic transformation efficiency have been developed in other crops by enhancing embryogenesis or shoot organogenesis through the expression of transcription factors like Wuschel2, Baby boom and GROWTH REGULATING FACTORs (GRFs)-GRF-INTERACTION FACTOR (GIF)[115]. Testing these technologies in key cassava cultivars could help overcome genotype-dependent constraints and further enhance transformation efficiency.
-
CRISPR/Cas9 has revolutionized genetic manipulation, transitioning from use in animal cell lines to becoming a pivotal tool in agricultural research and crop development. This technology is instrumental in enhancing crop productivity, quality, and nutritional value. With its unprecedented speed and precision, CRISPR/Cas9 simplifies mutant identification within large plant populations, significantly reducing the need for extensive screening. Its versatility allows targeting any DNA sequence with remarkable efficiency, often reaching 100%, making it the most powerful genome editing tool available. CRISPR/Cas9 has been applied to crucial breeding targets, including yield improvement, quality enhancement, herbicide resistance, and stress tolerance. Additionally, it enables the modification of plant traits such as inflorescence patterns, architecture, and gene expression through precise transcriptional regulation. Importantly, CRISPR/Cas9-induced mutations have been shown to be heritable across sexual generations, further amplifying its utility.
Beyond cassava, CRISPR/Cas9 technology has been successfully applied to a wide range of plant species, including Arabidopsis, tobacco, sorghum, rice, tomatoes, potato, populus, and cucumber[116]. However, the biosecurity of genome-edited plants remains a concern. The integration of CRISPR/Cas9 constructs into the plant genome via Agrobacterium tumefaciens-mediated T-DNA transfer can lead to unintended genetic changes and off-target effects. This is particularly challenging for perennial plants and vegetatively propagated crops like cassava, where removing CRISPR/Cas9 cassettes is difficult. As a result, achieving DNA-free genome editing through transient delivery of Cas9-encoding mRNA, guide RNA, or RNP into plant protoplasts is preferable.
Creating mutations in transformation-recalcitrant species, such as soybean, sorghum, cotton, and woody plants, remains technically challenging. Optimization strategies to improve transformation efficiency, including delivery methods and protoplast regeneration, are needed. Additionally, employing a co-editing strategy to generate transgene-free plants for breeding purposes shows great promise[117]. Despite its vast potential, CRISPR/Cas9 has limitations, such as off-target effects and the requirement for a nearby PAM sequence for editing target genes. Therefore, systematic evaluation of CRISPR/Cas9 specificity and the exploration of new methods, such as the nickase strategy, are essential to address these challenges.
This work was supported by the National Natural Science Foundation of China (32072118, 32160398), Central Public-interest Scientific Institution Basal Research Fund (NO. 1630052024001), and the Earmarked Fund for China Agriculture Research System (CARS-11).
-
The authors confirm contribution to the paper as follows: study conception and design: Zhang P, Ma Q; data collection: Tong W, Cheng L, Zhang Y, Wang Y, Wang X, Feng Y, Li R, Lu X, Yan W; analysis and interpretation of results: Zhang P; draft manuscript preparation: Ma Q, Zhang P. All authors reviewed the results and approved the final version of the manuscript.
-
All data generated or analyzed during this study are included in this published article.
-
The authors declare that they have no conflict of interest.
-
Received 19 September 2024; Accepted 5 December 2024; Published online 11 February 2025
- Copyright: © 2025 by the author(s). Published by Maximum Academic Press on behalf of Hainan University. This article is an open access article distributed under Creative Commons Attribution License (CC BY 4.0), visit https://creativecommons.org/licenses/by/4.0/.
-
About this article
Cite this article
Ma Q, Tong W, Cheng L, Zhang Y, Wang Y, et al. 2025. Advancing cassava molecular breeding through genome editing: a promising pathway. Tropical Plants 4: e004 doi: 10.48130/tp-0024-0046
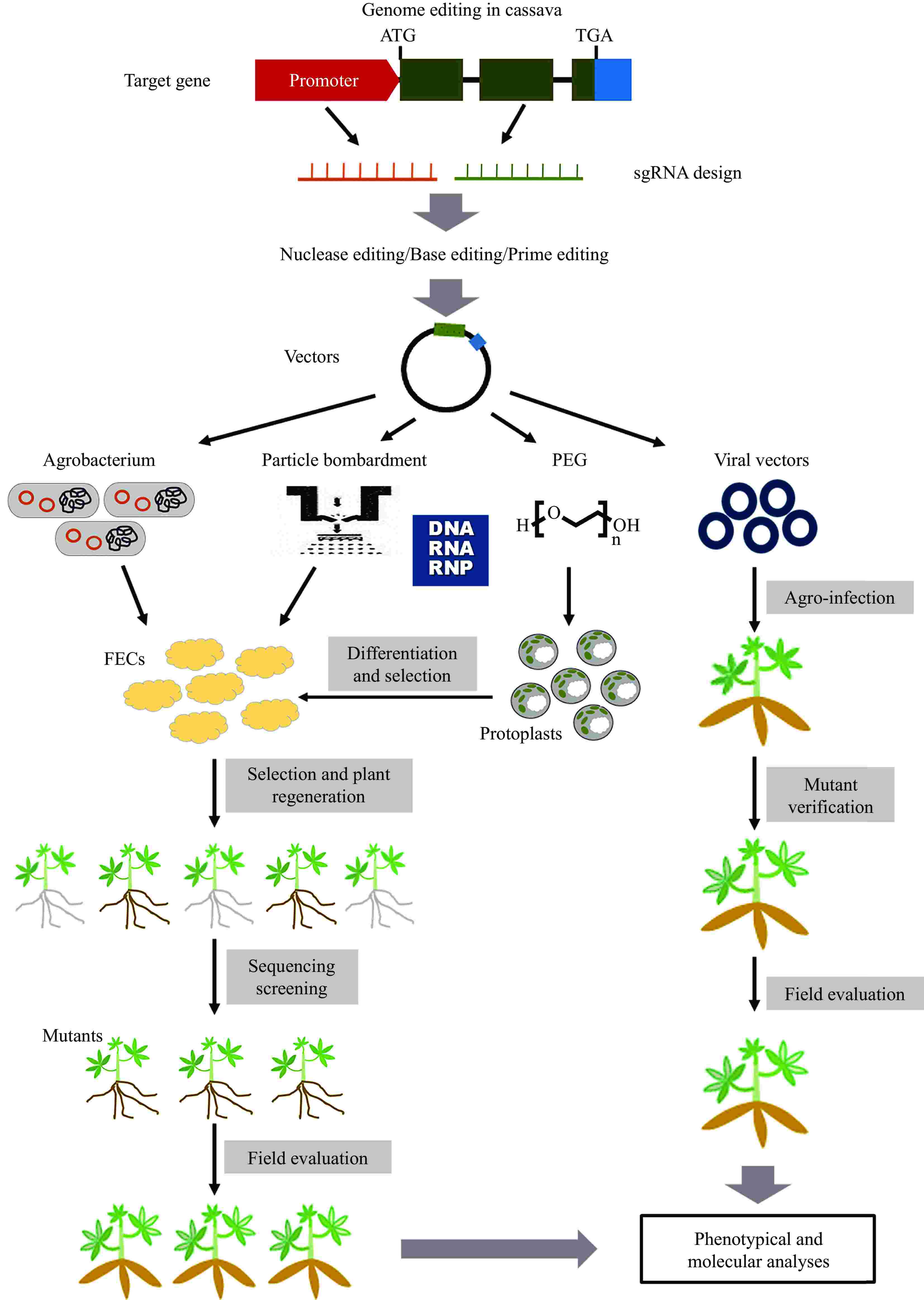