-
Seed plants, including gymnosperms and angiosperms, emerged 360 million years ago and are the highest evolutionary class of plants. Seeds are a key component of the plant life cycle and are also an important source of nutrients for humans and many animals. The seeds of angiosperms comprise of embryo, endosperm, and seed coat, which are developed from fertilized egg cells and central cells as well as maternal integument (testa), respectively. The diploid embryo and the triploid endosperm are two closely juxtaposed tissues that constantly develop and interact to form different types of seeds. During the middle and late stages of seed maturation, storage material accumulates in the embryo or endosperm. Additionally, the testa develops from the maternal ovule integument, and the seed coat not only determines the size of embryo and the endosperm development space but also provides protection for seeds. Unlike angiosperms, the endosperm of gymnosperms develops from female gametophytes before fertilization, which functions as a storage organ for seed nutrition. The gymnosperm zygote matures through the proembryo development stage, the early embryogenesis stage, and the late embryogenesis stage[1]. Dysplastic embryos, endosperms, or testae tend to result in abnormal seed size and morphology. Seed size and morphology are important traits associated with crop yield and plant fitness, so understanding the molecular mechanisms and regulatory networks during seed development is of great help in breeding high-yield crop varieties. As an adaptation of plants acquired during long-term evolution, seed dormancy can avoid germination under unfavorable environmental conditions to improve seedling survival and also prevent off-season germination to reduce competition between species. When plants perceive suitable external signals, seeds begin to enter the germination stage. Germination is an important stage of plant growth and development. At germination, the seeds begin to absorb large amounts of water until the seed coat breaks. Cell growth and expansion begin to occur after the rupture of the seed coat, which ultimately leads to the emergence of radicles. Phytohormones synergistically regulate these development processes in seeds.
Brassinosteroids (BRs), the sixth phytohormone, play an important role in seedling development, flowering and fruiting, seed development, and maturation by regulating cell proliferation and cell elongation[2]. Because BR biosynthesis and signal pathways have been well reviewed[3,4], the related components will not be repeatedly described here. Phytohormones such as ABA and GA play an important role in seed development[5], however, increasing evidence indicates that BRs are also an important player. The contents of two bioactive brassinosteroids, brassinolide (BL), and castasterone (CS), increased significantly during the development of pea seeds and decreased sharply after the seed size reached its maximum[6], suggesting that BRs may play an important role in seed morphogenesis. Probably due to the limitation of the low BR content and determination technique, coupled with the large amount of sample required for BR determination, dynamic changes in BR levels and species during seed development, as well as the distribution of BRs in embryos, endosperms, and seed coats in seeds have less been reported. BR analysis of 5-week Arabidopsis plants revealed that BL was not detected in stem and rosette leaf tissues, while the content of CS, teasterone (TE), and typhasterol (TY) in immature siliques was more than five times higher than that of rosette leaves, and also contained BL at approximately 0.2 ng/gfw[7]. Considering that the BR content may be lower in pods than in developing seeds, it is speculated that the level of active BRs in developing seeds may be much higher than that in other tissues, and the species may be richer, especially the presence of highly active BL, which may be essential for the rapid development of seeds. This speculation remains to be proved in more species.
Notably, as in the vegetative tissues of angiosperms, CS is mainly the active BR in lower land plants. Moreover, its content is several orders of magnitude lower than the CS content in seed plants, suggesting that BRs play an important role in seed plants[8]. Tomato CYP85A1 mutants showed severe BR defects in vegetative growth due to blocked CS synthesis in vegetative organs, but could still bear normal fruits. Moreover, the homologous gene CYP85A3 was expressed specifically in fruits, and the BL content in these fruits approximated that of wild type. This indicates that CS plays a major role in vegetative growth in tomatoes, while BL functions mainly in reproductive growth[9,10], suggesting that BL may play an important role in seed development. However, CS may be the predominant active BR in monocots like lower plants[11]. So far, there has been no report of BL in the model monocot rice. Whether CS can be further catalyzed to more active BL only in dicots, more evidence is needed. Because CS cannot form hydrogen bonds with tyrosine residue in the island domain of BR receptors Brasinosteroid Insensitive 1 (BRI1) binds to BL with greater affinity than CS, which may be responsible for the difference between BL activity and CS activity[12]. Because of the technical difficulty in determining the BR content, some questions remain open, for example: Are there differences between CS and BL in different tissues at different stages of plant seed development? Is the differentiation of monocot and dicot associated with BL? With technology improvements, less amount of samples can be used for the identification and quantitative analysis of BR species[7]. It is possible that these studies could be conducted, particularly when large seeds are used.
Given the limited reports on BR content in seeds, analysis of gene expression and protein levels related to BR synthesis and signaling in seeds could help understand BR function in seed development. Probably due to the relatively low abundance and detection power of proteins related to BR synthesis and signaling, we did not find significant clues when mining some published proteomics data. The results of transcriptome sequencing of embryos at different developmental stages suggest that BRs may play an active role in the embryo morphogenetic stage[13]. In rice, OsDWARF4 (OsDWF4) is stably expressed during the early stages of seed development and is enhanced during the later stages of 15−20 DAF[14]. Analysis of transcriptome data from Arabidopsis seed embryos at different stages[15] suggests that the expression of DWARF1 (DWF1) and DWF4 gradually increased in seed development, but decreased rapidly in embryos of mature green seeds. Arabidopsis CYP85A2 can catalyze the conversion of 6-deoxyCS to BL during BR synthesis[9]. However, the expression of CYP82A2, on the other hand, showed opposite changes to DWF1 and DWF4, suggesting that BL mainly plays its role at the early development stage. The expression of Brassinazole Resistant 1 (BZR1), BRI 1-associated kinase 1 (BAK1), and BRI1 EMS Suppressor 1 (BES1), the BR signal genes, increases at the beginning of the heart-shaped embryo stage and declines at the mature green seed stage. The expression of Brassinosteroid-Insensitive2 (BIN2) gradually increased as seed development progressed, which may be related to the continuous weakening of BR signaling as seed development progressed. BR degradation metabolism is an important factor in controlling BR content levels. Expression of BR metabolic genes, except for CYP734A1 (BAS1), was at low levels in the early stage of seed development and slightly increased in the later stage, suggesting that BR degradation was not active early in seed development. In summary, the abundant content of BRs in seeds and various signaling changes may mean that BRs play an active role in seed development.
BRs have emerged as early as in lower plants, such as mosses, lycophytes, and ferns[8]. BRI1, BRI1-like 2 (BRL2), and BRI1-like 1/3 (BRL1/3) are highly expressed in vascular plants such as ferns, gymnosperms, and angiosperms, respectively[16]. BRI1 is the most potent BR receptor in Arabidopsis, and BRL1/3 can partially replace the function of BRI1, while BRL2 is a BR non-functional receptor[17]. Whole-genome duplications optimize BR synthase and BR receptors by initiating a novel functionalization process differentiated by ligand sensing and transcriptional expression, which promotes the evolution of land plants, especially angiosperms[16].
BRs play an important role in seed development, dormancy, and germination. However, there is a lack of overall reviews on BRs and seed development. BRs are present in lower plants[8], suggesting that the BR signaling pathway derives and functions earlier than seed plants. However, the high content of BRs in seeds means that its role is greatly enhanced during seed development. So, is the mechanism of action of BRs in plant seed development similar to other tissues? Or is it an extension of its function in seeds? Because orthodox BR signaling originated before the emergence of angiosperms and after the separation of gymnosperms and angiosperms[18], is BR based on the needs of seed development and derives new mechanisms of action? To our knowledge, there is only one report of BR on gymnosperm seeds, in which BR can promote seed germination of conifers[19]. Therefore, this review focuses on recent research advances in the development, dormancy, and germination of angiosperm seeds related to BRs. Considering that the brassinosteroid regulation in rice was well reviewed[20], we will not repeat these reports and only update some new results on rice. Our opinion and some suggestions are put forward for further research.
-
The morphogenesis and maturation of seeds are two important stages of seed development. The seed morphogenetic stage begins with double fertilization, during which the seed embryo and endosperm will maintain highly coordinated cell division and differentiation until the basic morphology of the seed is established. During seed maturation, programmed cell death occurs in the endosperm of many dicotyledonous plants to nourish developing embryos, whereas in monocotyledonous plants, the endosperm remains in mature seeds to provide nutrients for seedling development. The accumulation of a large number of storage compounds such as starches, lipids, and proteins in the embryo or endosperm is also an important marker during seed maturation[21].
BRs can affect the division and expansion of embryonic cells during the morphogenetic stage of seed development and an abnormal BR content often leads to abnormal seed development. FACKEL encodes C-14 sterol reductase for BR biosynthesis and the mutant fk-J79 of FACKEL causes delayed embryonic development and disrupted cell division with normal apical-basal and lateral axes in seed development[22]. The det2 mutant similarly showed a delay in embryonic development and a reduction in the size and number of embryonic cells[23]. Furthermore, abnormalities in the BR signaling pathway can also lead to abnormal division of embryonic cells. For example, abnormalities in BRI-1 in barley lead to delayed grain development[24]. The maize ZmSK2, a GSK3-like kinase, is a homolog of Arabidopsis BIN2. Embryonic development of the ZmSK2-overexpressing line is impeded at the transition stage probably due to the phosphorylation of the IAA28 protein by ZmSK2, which leads to embryonic lethality of the homozygous line[25]. Dornroeschen (DRN) and its paralog Dornroeschen-Like (DRNL), AP2/ERF-type transcription factors, function in embryo cell division by interacting with BR signaling. Loss-of-function of the BES interacting Myc-like protein1 (BIM1) shows developmental defects in seed embryos, and the DRN, DRNL, and Phavoluta (PHV) complexes can regulate embryo morphology by interacting with BIM1[26], although the specific mechanism remains to be revealed. Somatic Embryogenesis Receptor-Like Kinases1 (SERK1) and SERK2, homologous proteins of BAK1, are essential for early embryonic development[27].
The coordinated development of embryo, endosperm, and seed coat is essential for normal seed development. BRs also regulate seed development by regulating endosperm and seed coat. The det2 mutant showed a reduced volume of endosperm[23]. Furthermore, rice lines of RNAi-silenced BAK1 also showed a severe delay in endosperm development[28]. Short Hypocotyl Under Blue1 (SHB1), Haiku1 (IKU1), and IKU2 play an important role in seed endosperm development. SHB1 is a positive regulator of seed development by regulating the expression of the Miniseeds3 (MINI3) and IKU2 related to endosperm development[29]. IKU1, expressed in the early endosperm and its ancestral central cells, interacts with MINI3 to form a complex to regulate the downstream gene IKU2 and positively regulates seed size by promoting endosperm development[30]. Moreover, BZR1 can bind to the promoters of SHB1, IKU1, and IKU2 and activate their expression, which are positively associated with seed size[23]. RAV1 negatively regulates MINI3 and IKU2, and RAV1-overexpressing lines all show abnormalities in ovule development, seed size, and seed weight[31]. RAV1 expression remains low during the early stages of seed development and increases dramatically during seed maturation. BES1 is able to directly bind to the RAV1 promoter and suppress its expression[32]. Although these studies revealed BR's role in endosperm development, the detail mechanism still needs to be elaborated.
INNER NO OUTER (INO), a YABBA family protein, plays an important role in seed coat development, and the growth of seed coats of the ino mutant is inhibited. BR can regulate seed coat development by regulating the expression of INO through BZR1[33]. Auxin Response Factor2 (ARF2) can regulate seed development by regulating seed coat growth, and its mutant mnt/arf2-9 shows promoted seed coat growth[34]. Apetala2 (AP2) plays an important role in the development of embryo, endosperm, and seed coat. The ap2 seeds exhibit delayed endosperm cellularization and overgrowth of the endosperm central vacuole, and seed coat cells are more elongated than wild type[35]. BZR1 has been shown to bind to the promoters of ARF2 and AP2 and repress their expression[23].
-
Plants produce carbohydrates by photosynthesis and transport them in the form of sucrose to tissues such as roots, stems, and seeds through the phloem to provide carbon and energy for plant growth. BRs also largely impact the accumulation of storage material during seed maturation. Overexpression of BR biosynthesis genes in rice and maize altered starch species and total protein and starch content in seeds. Rice DWARF11 overexpression lines showed a significant decrease in amylose content in seeds, but a significant increase in total starch and protein content[36]. Additionally, the expression of genes related to starch synthesis was regulated and the viscosity of flour was enhanced in overexpression lines of the BR biosynthesis gene DWF4 in rice[14]. The expression of BR signaling-related genes can also have an impact on starch accumulation in seeds. Amylose synthesis and accumulation were delayed in endosperm and amylopectin structure was altered in seeds of the barley bri1-1 mutant[24]. RNAi silencing of OsI-BAK1 in rice results in a large number of rudimentary green and unfilled grains, in which starch granules were not found in the cross-sections[28]. Carbon Starved Anther (CSA) plays a critical role in regulating sugar partitioning required for pollen and seed development. OsBZR1 can regulate sugar partitioning and starch synthesis during seed development by regulating CSA expression[37]. Furthermore, BR has been shown to promote seed weight by stimulating the flow of assimilates from the source to the sink, as well as the accumulation of starch in seeds, although this promotion was thought to be derived from increased BR activity in stems and leaves rather than the seeds themselves[38]. However, using seed-specific expression of BR synthesis genes and signaling elements in Arabidopsis and rice, this indirect effect of maternal BR activity on seed substance accumulation could be ruled out and a direct positive effect was confirmed in these transgenic seeds. In terms of seed oil accumulation, it has been shown that overexpression of AtDWF4 in rapeseed increased seed grain weight, but did not alter the relative oil content or fatty acid components of seeds[39]. However, exogenous 24-epibrassinolide (EBL) did promote fatty acid accumulation and alter fatty acid composition in seeds of Styrax tonkinensis[40]. Therefore, further research is needed to investigate whether BRs can change the oil content or fatty acid composition of seeds. The regulatory mechanism of BRs in seed development is summarized in Fig. 1.
Figure 1.
BR regulates seed development and accumulation of storage materials mainly through transcription factors BZR1 and BES1. In the regulation of seed coat development, BZR1 can bind to the promoter of ARF2, a negative regulator gene for seed coat development, and inhibit its expression, and BZR1 can bind to the promoter of INO, a positive regulator gene for seed coat development, and promote its expression. Additionally, AP2 negatively regulates seed coat, embryo and endosperm, and BZR1 can bind to the promoter of AP2 and inhibit its expression. In the regulation of seed endosperm development, BES1 can inhibit the expression of the negative regulatory genes RAV1 and ABI5, which are associated with endosperm development, to positively regulate seed endosperm development. BZR1 can bind to the promoters of positive regulators of endosperm development, SHB1, IKU1, IKU2, and activate their expression. In the regulation of seed embryo development, BES1 can promote the expression of KPP6 to positively regulate embryo development, and the BR signal transduction element BIM1 can also interact with DRN, DRNL, or PHV to regulate early embryonic development. Furthermore, the accumulation of oil, starch and protein in seeds can be affected by changes of BR content through up-regulation of BR-biosynthesis genes (DWF4 or DWF11) or exogenous 24-epibrassinolide. The component and accumulation of starch can be regulated by the BRI1 and BAK1 BR-signal. BZR1 can regulate sugar partitioning as well as starch synthesis through the regulation of CSA expression. Activated and repressive effects are shown by arrows and bars, respectively. Blue represents the BR and BR signaling components and red represents the ABA signaling component.
-
Seed size is a comprehensive result of the development of embryo, endosperm, and seed coat, and the accumulation of storage materials. We believe that the roles of BRs in seed morphogenesis and accumulation of storage materials described above may also directly or indirectly contribute to seed size, more or less. However, some studies only revealed the relationship between BR and seed size but did not unmask the specific mechanism of the effect, which could not be specifically discussed above. Because seed size is closely related to crop yield, here we discuss more reports from other species of angiosperm or new reports in rice.
BRs cannot be transported over long distances from one plant tissue to another. Therefore, maintaining the balance of active BR content within cells or tissues is essential for plants. Abnormal changes of BR content during seed development may lead to aberrant morphology or size of mature seeds. Seeds of rice dwarf11 mutants become smaller than the wild type[41]. Similarly, seeds of Arabidopsis det2 mutant are smaller and lighter than the wild type due to reduced endosperm volume and delayed embryonic development, and exogenous application of BR restored this abnormal phenotype to the wild type[42]. Additionally, OsCYP51H3 encodes obtusifoliol 14α-demethylase in the BR biosynthesis pathway, and its mutant exhibits much shorter seeds[43]. Overexpressing genes involved in BR biosynthesis often exhibit phenotypes with longer seeds and increased grain weight, such as seeds from AtDWF4, TaD11 overexpression lines[39,44]. Furthermore, some genes are able to regulate seed size by affecting endogenous BR content. For example, abnormalities in seed development of mutants of lon1-2 and atg5-1, two mitophagy-related genes, are associated with the significant down-regulation of expression of BR-biosynthesis genes[45].
BR metabolizing enzymes, which inactivate BR by modifying the BR structure, also play an important role in the homeostasis of active BR content in plant cells[4]. The Arabidopsis shk1-D mutant, with elevated expression of the BR metabolism-related gene CYP72C1, also showed a decrease in seed size[46]. The F-box-mediated proteolytic pathway plays an important role in the regulation of key functional proteins for plant growth and development. Inhibition of rice FBX206 expression, an ubiquitin ligase F-box E3 related to the proteolytic pathway, increased the expression of genes related to BR synthesis and decreased the gene expression of BR metabolizing enzymes in transgenic plants, and these factors together led to the accumulation of intermediate 28-homoBL BR synthesis in these lines and the increase in grain size[47]. In summary, BR synthesis and degradation regulate the level of BR accumulation, which positively regulates seed size.
Similarly, regulation of the BR signaling pathway also impacts seed size. Arabidopsis bri1-5 has a smaller seed size than wild-type seeds[42]. In addition, some proteins can regulate seed size by regulating BRI1. ZnF encodes an E3 ubiquitin ligase, which can ubiquitinate TaBKI1 and lead to the degradation of TaBKI1. ZnF knockout lines have been found to have reduced grains, which may be due to the inability of TaBKI1 degradation after ZnF deletion, resulting in abnormal binding of BAK1 to BRI1[48]. Moreover, Small Grain 3 (SMG3) and Decreased Grain Size 1 (DGS1) can influence seed size by mediating the degradation of misfolded or incompletely folded BRI1. The seed size decreased in loss-of-function mutants of SMG3 or DGS1 and the seed length increased in their overexpression lines[48]. This evidence suggests that BRI1 plays an important role in determining seed size. Glycogen Synthase Kinase-2 (GSK2), a homologous gene of Arabidopsis BIN2 in rice, can regulate seed size by regulating genes involved in seed development. The MAPK Kinase Kinase 10 (MAPKKK10)-MAPK Kinase 4 (MAPKK4)-MAPK6 cascade pathway controls seed size by regulating WRKY53[49,50]. GSK2 can regulate seed size by phosphorylating WRKY53 and MAPKK4. Furthermore, the interaction of WRKY53 and BZR1 promotes seed size[51]. Rice sg2 mutants exhibit a reduced grain size phenotype due to suppressed cell growth in spikelet shells, and there is evidence that SG2 is a downstream gene of GSK2 and functions as a positive regulator of BR signaling[52]. From these studies, it is concluded that BIN2 plays an important role in the regulation of seed growth and development, which in turn affects seed size. BZR1 and BES1/BZR2 are important transcription factors associated with plant growth, development, and reproduction in the BR signaling pathway. BZR1 and BES1/BZR2 are also believed to play an important role in seed development. The genome-wide association analysis of soybean suggests that BZR1 and BRI1 may play an important role in seed weight[53]. Association analysis of 513 candidate genes from different inbred lines in maize showed that SNPs associated with ZmBES1/BZR1-5 were closely related to grain width and grain weight[54]. Furthermore, rice OsBES1 haplotype analysis showed that all OsBES1s were associated with the development of grain size and could regulate grain weight, length, or width[55]. Overexpressing lines of BZR1 often increase seed size[56]. In rice, OsBZR1 can bind directly to the OsBAK2 promoter to repress its expression, and the grain length of mutant osbak2 increases, while the grain length of osbak2/osbzr1 decreases. This evidence suggests that OsBAK2 and OsBZR1 have a negative feedback regulation on the regulation of seed grain length[57]. OsPUB43 can regulate seed growth and development by inhibiting cell proliferation in the spikelet shell, while BZR1 can regulate seed size by activating OsPUB43 expression[58]. In addition, BES1 can regulate seed size by inhibiting ABA synthesis and signaling, which are negative regulators of seed size. ABA Insensitive 5 (ABI5) can directly repress SHB1, a positive regulator of seed development, leading to a decrease in seed size[59]. BES1 can bind to the promoter of ABI5 and repress its expression[60]. BES1 can also directly bind to the E-box sequence in the promoter of ABA2, a gene for ABA biosynthesis, to regulate seed size by decreasing endogenous levels of ABA in plants[60]. Seed-specific expression of AtBZR1 and AtBES1 can also increase seed size by down-regulating ABA signaling through inhibition of AtABA2 and AtABI5[61]. ICK/KRP family genes play an important role in cell division by affecting CDK[62]. GhBES1.4 has been shown to directly promote the expression of Kip-Related Protein6 (GhKRP6) by binding to the CACGTG motif in its promoter region. Notably, cotton GhKRP6 silenced lines have smaller seeds and reduced grain weight, which may be associated with the expansion of seed cells[63]. This evidence suggests that BR signaling affects seed size through multiple pathways (Fig. 2).
Figure 2.
BRs change morphology and size of seeds. Expression of the BR-synthesis genes DET2, DWF4, CPB1, and D11 can enlarge seeds by increasing the BR content. The expression of the BR-degradation gene CYP72C1 can reduce the BR content and thus reduce the seed size. Additionally, CYP51H3, Lon1, ATG5, FBX206 can also change seed size by affecting BR content. SMG3 as well as DGS1 can regulate seed size by degrading the aberrant BRI1 protein. ZnF changes seed size by degrading the BKI1 protein, prompting the binding of BRI1 to BAK1. BIN2 alters seed size by phosphorylating SG2, WRKY53, and MAPKK4, respectively. BES1 and BZR1 can change seed size by promoting the expression of KPP6 and BAK2, respectively. In addition, BZR1 and BES1 can enlarge seeds by inhibiting the expression of ABA-related ABI5 and ABA2. Activated and repressive effects are shown by arrows and bars, respectively. The blue and red letters represent the BR and BR signaling components and the ABA signaling components, respectively. The colors of the background represent the components of a certain plant, e.g., yellow represents rice. To be concise, other factors affecting seed size in rice[20] are not presented here.
-
It is well known that seed dormancy and germination are largely affected by the GA and ABA pathways during the maturation stage. A growing body of research suggests that BRs also play an important role in dormancy and germination. The rapid decrease of BR content at the seed maturity stage hints at its role in dormancy. Delay of Germination-1 (DOG1) is associated with seed dormancy. The expression of BR-related genes increases dramatically in the dog1 mutant, suggesting that repressing the expression of BR-related genes may be an important aspect of DOG1 in regulating dormancy[64]. During seed germination, the BR content and expression of some BR-related genes increase. For example, the content of CS increases during the germination of pea seeds, and the contents of BR-synthesis intermediates, including 6-deoxoCS, 6-deoxotyphasterol (6-deoxoTY) and 6-deoxo-3-dehydroteasterone (6-deoxo3DT), also increase[6]. The expression of BR-related genes, such as CYP85A1, BAK1, BZR1, and BRI1, increases during potato germination[65]. Furthermore, seed germination is delayed in both the BR biosynthesis mutant dwarf11 and the BR-insensitive mutant bri1-5[66,67]. Transcriptome and chromatin immunoprecipitation microarray (ChIP-chip) data also indicate that BZR1 can directly target many seed germination-related genes, such as Repressor GA (RGA) and Phytochrome B[68]. These evidences suggest that BRs may also play an important function in seed germination regulation.
A large number of studies have shown that ABA antagonism with GA regulates seed dormancy and germination. Further studies have shown that BRs affect dormancy and germination by cross-talking with the ABA and GA pathways. For example, BRs can act synergistically with GA to break seed dormancy and promote seed germination. The germination rate of Arabidopsis GA-synthesis mutant ga1-3 was reduced, and the application of GA or exogenous BR could restore the germination of ga1-3[69]. Furthermore, exogenous application of 1 μM GA could rescue delayed germination of bri1-5 seeds, and application of paclobutrazol, a GA biosynthesis inhibitor, inhibited germination of BRI1-overexpressing seeds[67]. These results indicate that BRs can positively regulate GA signaling to break dormancy and promote germination. Furthermore, BRs can be involved in seed germination by regulating GA biosynthesis-related genes and GA signaling pathway-related genes. The DELLA protein RGL2 is a major inhibitor of seed germination up-regulated by exogenous application of BR[70]. It has been shown that BR is located upstream of the GA synthesis gene GA20ox1, so the exogenous application of BR can break seed dormancy by promoting GA biosynthesis[71]. Additionally, cold stratification is a common means of breaking seed dormancy. Seeds of bri1-5 mutant were significantly less sensitive to the release of dormancy from cold stratification. Unlike wild type, cold stratification did not lead to up-regulation of GA biosynthesis genes in bri1-5. This evidence suggests that the BR signal plays an important role in releasing dormancy in cold stratification and the BRI1 is upstream of GA biosynthetic genes in cold stratification[67]. BRs are also capable of improving GA-induced seed germination by modulating the heterotrimeric G protein Galpha 1 (GPA1)[72]. Brassinosteroid Enhanced Expression 2 (BEE2) and Homolog of Bee2 Interacting with IBH1 (HBI1) are important proteins associated with seed germination, and BR and GA can simultaneously promote the expression of HBI1 and BEE2. HBI1 and BEE2 promote cell elongation in the hypocotyl – radicle transition zone by activating GA-Stimulated Arabidopsis 6 (GASA6) expression, which leads to the promotion of seed germination[73]. Moreover, some factors can regulate seed germination by moderating both GA and BR-related genes. For example, heterotrimeric G-protein-coupled receptor 1 (GCR1) mutant gcr1 is less sensitive to GA and BR during seed germination. Additionally, GA can also positively regulate BR biosynthesis and signaling pathways. DELLA protein RGA can interact with BZR1 protein and inhibit its binding ability to the promoter of downstream genes, while exogenous GA can degrade DELLA protein, thereby restoring the regulatory ability of BZR1 protein[74]. Furthermore, GA can restore aberrant BR biosynthesis or signaling by inhibiting Late Embryogenesis Abundant (LEA33) expression in rice, thus promoting seed germination[75]. Although the roles of GA and BR in seed germination have been investigated, the molecular mechanisms by which GA and BR induce seed germination together have not been well elucidated.
ABA positively regulates seed dormancy and negatively regulates seed germination, while BRs can regulate seed dormancy and germination in a mutually antagonistic manner with ABA. In the regulation of dormancy, BIN2 may promote dormancy by enhancing ABA signaling, and ABA can also promote dormancy by inhibiting the positive regulatory signal of BRs. ABI5 is a negative regulator of seed germination and a positive regulator of seed dormancy. BIN2 can inhibit seed germination by phosphorylating ABI5. However, the regulation of dormancy by the BIN2-ABI5 cascade at seed maturity has not been elucidated. Additionally, Snakin-2 (StSN2) is associated with disease resistance in potato. It has been shown that StSN2 promotes dormancy in potato tuber because StSN2 and StBIN2 can interact and increase StBIN2 activity, which leads to inhibition of BR signaling and increased expression of the ABA-related genes StSnRK2.2/2.3/2.4/2.6 and StABI5[76]. However, whether BRs play an important role in seed dormancy remains to be further investigated.
In seed germination, BRs can alleviate the inhibitory effect of exogenous application of ABA, and this mitigation was more pronounced with higher BR concentrations applied[77]. Increased expression of DWF4 could also reduce the inhibition of seed germination by ABA[78]. Seeds of mutants det2 and bri1-1 germinate slowly and are sensitive to ABA, and this slow germination could be restored to normal by the ABA inhibitor fluridone[69,79]. Some evidence suggests that BRs can overcome ABA inhibition of germination through the regulation of BR-related genes. ABA Insensitive 1 (ABI1) and ABI2 are negative regulators of the ABA signaling pathway. ABI1 and ABI2 can dephosphorylate BIN2 and inactivate it, thus promoting the BR response, while exogenous ABA can mitigate this inhibition[80]. Additionally, the promoting effect of BRs on seed germination is achieved through the regulation of the ABA signaling pathway. ABI3 and ABI5 also play a critical role in ABA-mediated seed development. BES1 can form BES1-TOPLESS (TPL) - HDAC isoform (HDA19) co-repressor with TPL and HDA19 to deacetylate ABI3 chromatin histones, leading to transcriptional repression of ABI3[77]. In seed germination, BR can participate in the inhibition of ABI5, a negative regulator of seed germination, by various approaches. First, ABI5 is directly regulated by BZR1[81]. In apples, MdBZR1 can bind directly to the MdABI5 promoter and repress its expression, thus attenuating the inhibition of seed germination through ABI5[82]. Second, ABI5 can interact with BES1 in vitro and in vivo and be significantly inhibited from binding to the promoter region of its downstream genes, which promotes seed germination[83]. Third, ABI5 can be phosphorylated and stabilized by BIN2, thereby inhibiting seed germination, while BR can inhibit the BIN2-ABI5 cascade to antagonize ABA-mediated inhibition[84]. Similarly, BAK1 is also involved in the inhibition of ABA signaling in seed germination. Seeds of the bak1-4 abi1-1 double mutant were more sensitive to ABA than the abi1-1 mutant, suggesting that BAK1 is a negative regulator of ABA-inhibited seed germination[85].
During germination, starch acts as a major source of carbohydrates in seeds, providing energy for radicle elongation and subsequent seedling development. α- amylase plays a role in the degradation of starch to glucose during seed germination and repression of its expression significantly delayed seed germination[86]. Studies have shown that BZR1 can bind to the E-box at the promoter of α-amylase Rice α-Amylase gene (RAmy3D) and positively regulate its expression, which can promote starch degradation and the soluble sugar content during seed germination, thus promoting seed germination[87]. In addition to starch, proteins in the endosperm play critical roles in germination as nitrogen sources. Rice REP-1 encodes a cysteine protease for the degradation of gluten, a seed storage protein. BR and GA can induce the expression of REP-1 during seed germination to degrade gluten in endosperm and provide amino acids for embryo growth[88]. This evidence suggests that BR and GA can positively regulate seed germination by promoting starch and gluten degradation, while ABA does the opposite. The associated mechanisms of BRs in seed maturation and germination are summarized in Fig. 3.
Figure 3.
BRs regulate seed dormancy and germination mainly by cross-talking with components of ABA and GA signal. BR and GA can promote the expression of some genes to positively regulate seed germination, such as REP-1, HB1, BEE2, and the application of BR also inhibits the expression of the GA-related signal RGL2. BES1 can form BES1-TPL-HDA19 co-repressor and deacetylate ABI3 chromatin histones, repressing its transcription. Additionally, BIN2 can phosphorylate ABI5 and stabilize ABI5 protein, thus inhibiting seed germination. BZR1 is capable of binding to the upstream promoter of ABI5 and repressing ABI5 expression. BES1 is able to interact with ABI5 and inhibited binding of ABI5 to the promoter region of downstream genes, resulting in the promotion of seed germination. BZR1 can also promote the expression of α-amylase RAmy3D to positively regulate seed germination. In addition, ABA-related signal elements ABI1 and ABI2 can interact with BIN2 and dephosphorylate and inactivate BIN2, thereby promoting BR response. The GA-related signal element RGA can also inhibit BZR1 expression to repress the progression of the BR response. Activated and repressive effects are shown by arrows and bars, respectively. Blue represents BR and BR signaling components, green represents GA and GA signaling components, and red represents ABA signaling components. Dashed lines represents an indirect action.
-
The BR content in lower plants is significantly lower than in seed plants, suggesting an important role of BRs during the evolution of seed plants. Gymnosperms and angiosperms could have specialized on BR receptors during evolution, and BRI1 with higher binding capacity to BL could have emerged in angiosperms. Moreover, monocots and dicots could have diverged on the presence or absence of highly active BL. BL is found in reproductive tissues of dicots, including fruits, siliques, seeds, and pollens, while CS, TE, and TY rather than BL are identified in vegetative tissue, suggesting an important role of BL on the reproduction of dicots. To confirm this issue, the content and species of BRs should be determined in seeds of more species of gymnosperms and angiosperms including monocots and dicots. Furthermore, in dicots, we need to further investigate whether there are differences in BR species and activities between vegetative growth stages and seed development stages. Because the active components of BRs in seeds are different from other tissues, it is necessary to further study the BR synthesis and metabolic pathways in seeds of different plants.
BRs can regulate plant morphology through cell wall expansion, cell elongation, as well as cell division. The above reports suggest that BRs regulate seed development as well as seed morphology similarly to other tissues, which is also achieved through the BR signal elements, such as BIN2, BES1, and BZR1, to regulate genes related to seed development. The promoting effect of BRs on seed size is also similar to the effect of BRs on root length, and leaf size. Additionally, the function of BR in synergizing GA and antagonizing ABA to regulate seed dormancy and germination is not unique in seeds. For example, BR and ABA antagonize the regulation of stomatal closure, and BR and GA co-regulate photomorphogenesis. Therefore, the mechanism of BRs on seed development, dormancy, and germination was similar to other tissues, and a new mechanism of action in seeds has not been reported. Although BRs have been well studied in seed development, a large number of questions remain to be answered. For example: How can we strongly initiate BR synthesis and signaling pathways in zygotic embryos? How do we finely regulate BR synthesis and signaling pathways in different processes of embryo development to fit with these developmental processes? Why are high levels of BRs needed in seeds? How much do BRs contribute to the accumulation of seed storage materials and what are their special mechanisms? Therefore, there is a need for more detailed studies on the dynamics of BR content in different developmental stages, and if possible, analyzing the distribution of BRs in different tissues or cells. Additionally, single-cell transcriptomes during seed development and further related genetic studies may be helpful for fully understanding the biological functions of BRs in seed species.
Given these biological functions of BR, current knowledge can be applied to the improvement of the following seed traits: 1) Increasing grain weight/size, which can not only benefit the yield for human nutrition but also improve the basis for strong seedlings; 2) Changes of nutrition composition, such as an increase of protein content; 3) Modification of seed dormancy and germination. In carrying out these applications, both BR biosynthesis and signal pathways can be applied. In addition, the following issues may need to be considered: 1) A process of seed development. Such as increasing the BR level at the morphogenesis stage can promote cell division, which increases the sink volume. Reducing BR level at seed maturity was helpful to promote seed dormancy, which may be helpful for dealing with pre-harvest germination in warm and humid areas. 2) Tissues of seeds. For example, the oil mainly exists in the rice embryo; 3) Co-regulation with other hormone levels. Such as the synergistic effect with GA at the morphogenetic stage and seed germination stage, and the antagonistic function with ABA at the seed maturity stage. In general, BR regulation needs to be precisely controlled in these applications, which may be achieved by tissue-specific promoters or inducible promoters. For example, seed-specific overexpression of BR biosynthesis genes can significantly improve yield and quality[14,89]. With an in-depth understanding of BR functions, more concrete solutions can be proposed for the improvement of seed traits.
This work was supported by the National Natural Science Foundation of China (32171837) . We apologize to all authors whose important work is not cited due to limited space.
-
The authors confirm contribution to the paper as follows: study conception, manuscript and figures preparation: Chen A, Zhang M; manuscript and figures revision: Wang X, Zhang M. All authors reviewed the results and approved the final version of the manuscript.
-
The datasets generated during and/or analyzed during the current study are available from the corresponding author on reasonable request.
-
The authors declare that they have no conflict of interest.
- Copyright: © 2025 by the author(s). Published by Maximum Academic Press on behalf of Hainan Yazhou Bay Seed Laboratory. This article is an open access article distributed under Creative Commons Attribution License (CC BY 4.0), visit https://creativecommons.org/licenses/by/4.0/.
-
About this article
Cite this article
Chen A, Wang X, Zhang M. 2025. Do brassinosteroids enhance and upgrade their regulation roles in seeds? Seed Biology 4: e002 doi: 10.48130/seedbio-0025-0002
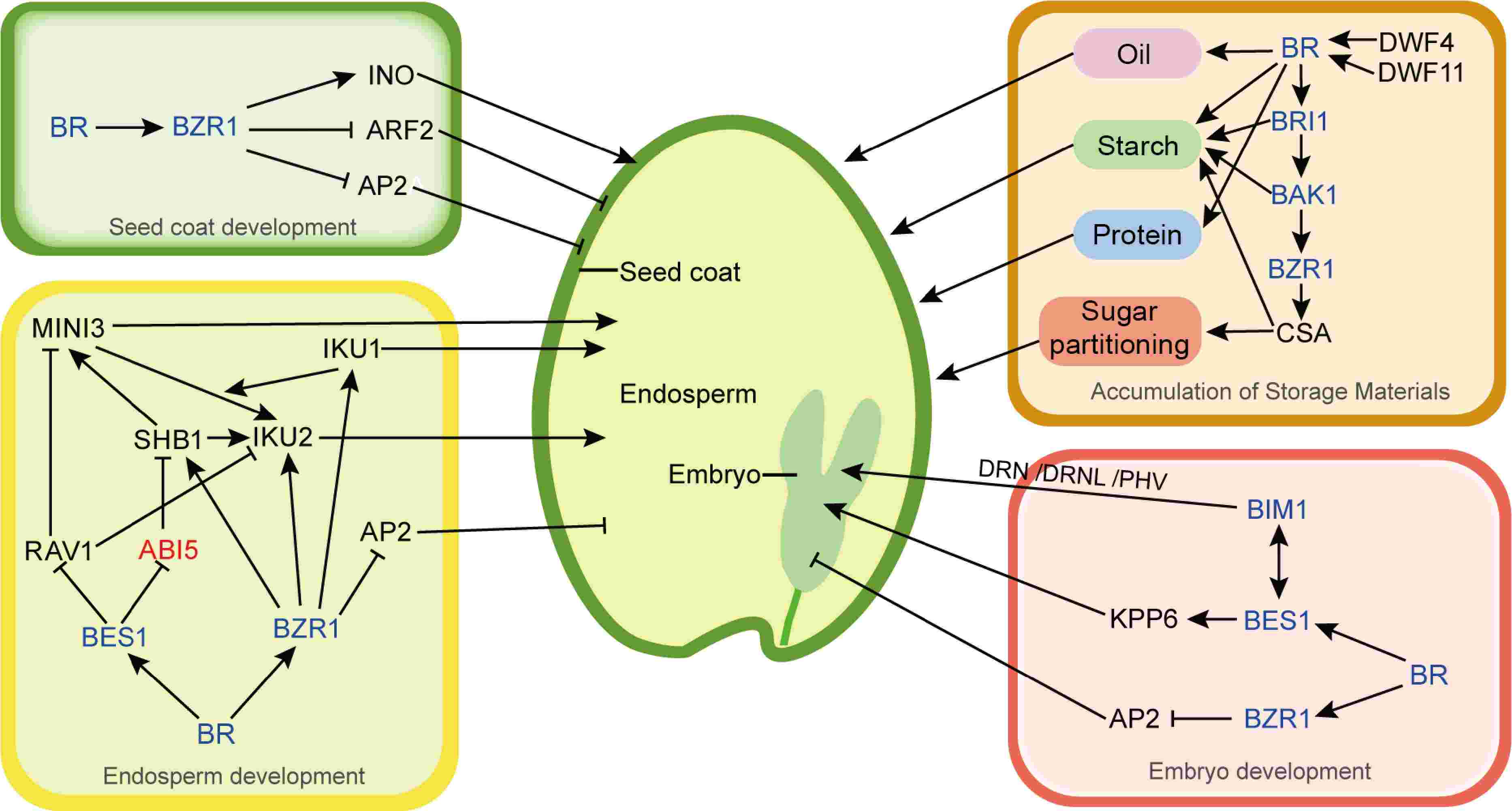