-
Plant growth and development depends on a constant supply of nutrient elements, since those essential elements exhibit a range of roles in providing nutrition for various processes, such as protein synthesis, enzyme activation, and osmotic adjustment, as well as participation in signaling perception and transduction during plant stress responses[1,2]. However, abiotic stresses, including heat stress, interrupt nutrient uptake, transport, and availability to plants with either nutrient deficiency or toxicity, adversely affecting plant growth[3]. Therefore, any approaches such as molecular priming leading to the maintenance of sufficient nutrients of beneficial elements and minimizing or avoidance of excessive accumulation of toxic ions are critical important for plant stress adaptation[4].
γ-aminobutyric acid (GABA) is a non-protein amino acid that is widely used as a molecular priming agent as it plays a positive role in regulating plant stress tolerance[5,6]. Improved plant tolerance to heat stress-induced by GABA-priming has been associated with up-regulating stress-defensive genes and antioxidant defense systems, as well as up-regulation of carbon metabolism and synthesis of various organic metabolites[7,8]. Exogenous application of GABA resulted in increased content of endogenous GABA, maltose, valine, and glutamate in creeping bentgrass under heat or drought stress[9−11], proline in black cumin (Nigella sativa) and peach (Amygdalus persica) fruit under drought and chilling stress[12,13], and trehalose in rice (Oryza sativa) under heat stress[8]. Recent studies have found that the GABA is not just a metabolite involved in the synthesis of other organic compounds, but may also effect ion availability in plant tissues, as it can directly regulate the activity of plant-specific anion transporters and mineral element transport by modifying membrane potential[14]. GABA also affects the transport of sodium (Na), potassium (K), and calcium (Ca) and reduces Na uptake which contributes to its positive effects to plant tolerance to salt stress[15]. GABA-primed plants of creeping bentgrass (Agrostis stolonifera) with improved salt tolerance had lower expression of genes controlling Na transport in leaves, including AsNHX1 and AsNHX4 (sodium hydrogen exchanger), AsSOS1 (salt sensitivity), and AsHKT4) (sodium transporter)[16]. Elevated GABA levels in plant tissues also reduces cytosolic pH due to the consumption of protons by converting glutamate to GABA[17]. The regulation of GABA to ion transporters and cytosolic pH levels might affect mineral nutrient availability or accumulation within plant tissues, and influence nutrient availability or accumulation in plants. However, the regulatory effects of GABA on inorganic nutrition associated with GABA-enhanced heat tolerance largely remain unknown.
The objectives of this study were to investigate whether GABA could alleviate heat stress damage involving the regulation of mineral nutrition in leaves and to identify major GABA-responsive nutrient elements contributing to GABA-improved heat tolerance in creeping bentgrass, a cool-season perennial grass species widely cultivated as turfgrass.
-
Heat stress suppressed shoot growth of creeping bentgrass whereas GABA application enhanced shoot growth under heat stress with increased endogenous GABA content (Fig. 1a, b). Leaf electrolyte leakage (EL) increased in response to heat stress, but GABA-treated plants exhibited 17% lower EL than non-treated plants under heat conditions (Fig. 1c). High temperature significantly reduced the content of chlorophyll (Chl), photochemical efficiency (Fv/Fm ratio), and net photosynthetic rate (Pn) of leaves after prolonged stress (Fig. 2a, b). Under the control temperature conditions, GABA application had no significant effects on Chl content, Pn, and Fv/Fm (Fig. 2a−c). GABA-treated plants maintained significantly higher Chl content, Pn, and Fv/Fm ratio than non-treated plants under heat stress (Fig. 2a−c). Heat stress led to significant declines in relative water content (RWC) and osmotic potential (OP) regardless of GABA application (Fig. 3a, b). Plants treated with GABA had 10% higher RWC and 15% lower OP than the non-treated control plants under heat stress (Fig. 3a, b).
Figure 1.
Effects of γ-aminobutyric acid (GABA) on (a) phenotypic change, (b) endogenous GABA content, and (c) electrolyte leakage (EL) in non-stress control plants and heat-stressed plants at 30 d of treatment in creeping bentgrass. Vertical bars represent standard errors of the mean (n = 4). Columns marked with different letters indicate significant differences between treatments (P < 0.05). C, control; C+G, control treated with 0.5 mM GABA; H, heat stress; H+G, heat-stressed plants treated with 0.5 mM GABA.
Figure 2.
Effects of γ-aminobutyric acid (GABA) on (a) chlorophyll (Chl) content, (b) net photosynthetic rate (Pn), and (c) photochemical efficiency (Fv/Fm) in non-stress control plants and heat-stressed plants at 30 d of treatment in creeping bentgrass. Vertical bars represent standard errors of the mean (n = 4). Columns marked with different letters indicate significant differences between treatments (P < 0.05). C, control; C+G, control treated with 0.5 mM GABA; H, heat stress; H+G, heat-stressed plants treated with 0.5 mM GABA.
Figure 3.
Effects of γ-aminobutyric acid (GABA) on (a) relative water content (RWC) and (b) osmotic potential (OP) in non-stress control plants and heat-stressed plants at 30 d of treatment in creeping bentgrass. Vertical bars represent standard errors of the mean (n = 4). Columns marked with different letters indicate significant differences between treatments (P < 0.05). C, control; C+G, control treated with 0.5 mM GABA; H, heat stress; H+G, heat-stressed plants treated with 0.5 mM GABA.
Macronutrients and micronutrients as affected by GABA priming
-
Heat map showed changes in 12 different nutrient elements in leaves in response to exogenous GABA application under normal conditions and heat stress (Fig. 4a). Total macronutrient element content decreased in response to heat stress, but GABA-treated plants maintained significantly higher total macroelement than untreated plants under non-stress conditions (Fig. 4b). GABA application did not have significant effects on total microelement content under heat stress (Fig. 4c).
Figure 4.
(a) Heat map of changes in 12 nutrient element levels. (b) Effects of γ-aminobutyric acid (GABA) on the total macronutrient element content (%), and (c) total micronutrient element content in non-stress control plants and heat-stressed plants at 30 d of treatment in creeping bentgrass. Vertical bars represent standard errors of the mean (n = 4). Columns marked with different letters indicate significant differences between treatments (P < 0.05). C, control; C+G, control treated with 0.5 mM GABA; H, heat stress; H+G, heat-stressed plants treated with 0.5 mM GABA.
The content of nitrogen (N), phosphorus (P), K, and Na in leaves decreased under heat stress regardless of GABA treatments (Fig. 5). Plants with GABA application showed significantly higher N, P, and Na content than those plants without GABA application under heat stress (Fig. 5a, b & f). GABA application promoted K accumulation under normal temperature conditions but not under heat stress (Fig. 5c). GABA-treated plants also exhibited significantly higher Ca content but lower magnesium (Mg) content than non-treated plants under heat stress (Fig. 5d, e). As shown in Fig. 6, copper (Cu), manganese (Mn), boron (B), zinc (Zn), and aluminum (Al) content did not show a significant difference between GABA-treated and non-treated plants under non-stress conditions. The GABA treatment significantly increased Cu content under heat stress, while GABA-treated plants showed significantly lower Mn and B content than non-treated control plants under heat stress (Fig. 6a, c & d). The content of iron (Fe), Zn, and Al was not changed due to GABA application under heat stress (Fig. 6b, e & f).
Figure 5.
Effects of γ-aminobutyric acid (GABA) on the content of (a) nitrogen (N), (b) phosphorus (P), (c) potassium (K), (d) calcium (Ca), (e) magnesium (Mg), and (f) sodium (Na) in non-stress control plants and heat-stressed plants at 30 d of treatment in creeping bentgrass. Vertical bars represent standard errors of the mean (n = 4). Columns marked with different letters indicate significant differences between treatments (P < 0.05). C, control; C+G, control treated with 0.5 mM GABA; H, heat stress; H+G, heat-stressed plants treated with 0.5 mM GABA.
Figure 6.
Effects of γ-aminobutyric acid (GABA) on the content of (a) copper (Cu), (b) iron (Fe), (c) manganese (Mn), (d) boron (B), (e) zinc (Zn), and (f) aluminum (Al) in non-stress control plants and heat-stressed plants at 30 d of treatment in creeping bentgrass. Vertical bars represent standard errors of the mean (n = 4). Columns marked with different letters indicate significant differences between treatments (P < 0.05). C, control; C+G, control treated with 0.5 mM GABA; H, heat stress; H+G, heat-stressed plants treated with 0.5 mM GABA.
The principal component analysis (PCA) of 12 detected elements in response to heat stress and GABA is shown in Fig. 7a. The first principal component (X-axis) or second principal component (Y-axis) accounted for 68.2% or 16.8% of the variance, respectively. Based on PCA analysis, Mg, B, N, Na, P, and Zn content were most responsive to exogenous GABA and heat stress (Fig. 7a). Figure 7b further shows that N, P, Ca, Na, or Cu was significantly up-regulated by GABA, while B, Mn, or Mg content was significantly down-regulated by GABA application under heat stress.
Figure 7.
(a) Principal component analysis (PCA) based on 12 different nutrient elements that were responsive to heat stress and exogenous γ-aminobutyric acid (GABA) and (b) significant effects of GABA on eight different nutrient elements that were responsive to heat stress (HG vs. H) in leaves of creeping bentgrass (the size of the circle indicates the degree of increase or decrease). H, heat stress; HG, heat-stressed plants treated with 0.5 mM GABA.
-
Previous research has mainly attributed promotive effects of GABA on plant tolerance to abiotic stress, including heat stress to the regulation of physiological and biochemical processes, as discussed in the Introduction, although stresses also interrupt nutrient uptake and metabolism[18], which could be modulated by GABA. In the current study, the improved heat tolerance in creeping bentgrass by GABA, as demonstrated by the maintenance of higher RWC, Chl content, Pn, and Fv/Fm, as well as lower OP and EL, could be associated with alteration of nutritional status and balance.
There is growing evidence to suggest that GABA is involved in regulation of nutrient transport in plants[5,14,19]. The analysis of combined transcriptomics and metabolomics suggested that GABA exhibited positive roles in maintenance of carbon (C)-N balance in Arabidopsis under C- and N-limited conditions[20]. Similar findings were observed regarding function of GABA on mediation of N uptake and utilization in Arabidopsis seedlings at low
media[21]. Foliar supplementation of N and P promoted heat tolerance in creeping bentgrass[22]. In this study, GABA application enhanced N and P content in leaves under heat stress, suggesting that GABA may enhance N and P supply to leaves contributing to GABA-improved heat tolerance.NO−3 Ca acts as an important regulator when plants suffer from multiple abiotic stresses due to its role in signaling transduction and osmotic adjustment among various other functions[23−25]. Foliar application of Ca significantly enhanced heat tolerance of Kentucky bluegrass (Poa pratensis) and tall fescue (Festuca arundinacea) associated with better antioxidant activities and water relations in leaves[26]. Appropriate supplements of Ca significantly improved the survival rate of wheat under heat treatment, whereas Ca inhibitors eliminated the positive effects of Ca[27]. Moderate Na accumulation is also closely related to osmotic adjustment and antioxidant ability in plants contributing to drought, cadmium, and salt tolerance[28−32]. Exogenous application of a low dose of Na effectively alleviated drought stress damage associated with improved water transport and balance in white clover (Trifolium repens)[33]. Previous findings proved that exogenous addition of GABA could increase Ca and Na absorption accompanied by growth promotion in leaves of duckweed (Lemna minor) under nutrient-deficient conditions[14]; exogenous GABA regulated Ca influx in tobacco pollen tubes to modulate pollen tube growth[34]. However, limited research information is available about the effects of GABA on Ca and Na in plants under heat stress. In the current study, plants with GABA application maintained significantly higher Ca and Na content than untreated plants under heat stress, which could be associated with the increase in osmotic adjustment and maintenance of better leaf hydration status in creeping bentgrass exposed to heat stress.
Micronutrient effecting on plant growth and stress tolerance requires delicate balance, as insufficient and over-accmulation can both be detrimental[35]. As an essential element, Cu exhibits multiple roles in biological processes and stress adaptability in plants, such as an important component of superoxide dismutase for antioxidant, the synthesis of Cu protein for photosynthesis, and a participator of N metabolism for N fixation[36,37]. Cu deficiency affected plant growth and normal physiological metabolism such as a decrease in photosynthesis[38−40]. In the present study, Cu content decreased with heat stress but was increased due to GABA application, which indicated that Cu could be involved in GABA-regulated adaptation to heat stress in creeping bentgrass. Alternatively, the overaccumulation of some micronutrients, such as B and Mn may have negative effects on stress tolerance for plants because these trace elements play their full roles at extremely lower levels[1,41,42]. In the current study, B and Mn content was reduced by exogenous GABA application, implying that GABA could mitigate potential B and Mn toxicity induced by heat stress, thereby alleviating heat stress damage in creeping bentgrass.
-
Priming of plants with GABA with increased endogenous GABA accumulation enhanced plant tolerance to heat stress in creeping bentgrass, as demonstrated by better leaf cellular hydration, membrane stability, and active photosynthesis. GABA priming-improved heat tolerance was associated with the accumulation of N, P, Ca, Na, and Cu and suppression of potential toxic B and Mn in leaves under a prolonged period of heat stress. Potential mechanisms of how GABA directly or indirectly affects nutrient uptake or transport deserve further investigation.
-
Creeping bentgrass (cv. Penncross) sods were collected from the turfgrass research farm at Rutgers University and transplanted into polyvinyl chloride tubes (30 cm in length, and 10 cm in diameter) filled with soil. Plants were grown in a greenhouse where the day/night temperature averaged at 23/16 °C and 790 μmol m−2 s−1 photosynthetically active radiation (PAR). Plants were cut twice per week to maintain a canopy height of approximately 4 cm and fertilized weekly with full Hoagland's solution[43]. After 40 d of transplanting, plants were moved to growth chambers controlled at 21/19 °C (day/night) and 12-h photoperiod with PAR of a 660 μmol m−2 s−1 at the canopy level.
After being acclimated for one week in growth chambers, plants in each tube were sprayed with 10 ml of 0.5 mM GABA solution or water (untreated control) every other day three times and then subjected to optimal growth temperature (control) (21/19 °C, day/night) and heat stress (35/30 °C, day/night) for 30 d. Each treatment was replicated four times (four containers) in four growth chambers.
Measurements of endogenous GABA and physiological parameters
-
For determination of endogenous GABA content, fresh leaves (0.2 g) were ground into a fine powder in liquid nitrogen, and 400 μM methanol was added. The mixture was vacuum dried. After adding 1 ml of 70 mM lanthanum chloride, samples were shaken for 15 min and centrifuged for 5 min at 13,000 g. The supernatant (0.5 ml) was then mixed with 160 μl of 1 M KOH and shaken for 5 min. The mixture was centrifuged again at 13,000 g to obtain the supernatant for GABA detection. One millilitre of reaction solution contained 0.1 ml of GABA extraction, 150 μl of 4 mM NADP+, 50 μl of GABA transaminase (2 units per ml), and 200 μl of 0.5 M K+ pyrophosphate buffer (pH 8.6). The initial absorbance of the reaction solution was read at 340 nm with a spectrophotometer (Spectronic Instruments, Rochester, NY, USA). Then 50 μl of 20 mM α-ketoglutarate was added into the reaction solution and the final absorbance was recorded at 340 nm[44].
Leaf RWC, OP, and Chl content were measured by using the method of Barrs & Weatherley[45], Blum[46] and Amnon[47], respectively. For photochemical efficiency, leaves were inserted in clips and kept in the dark for 15 min, and then a fluorescence meter (Fim 1500, Dynamax, Houston, TX, USA) was used to record variable fluorescence (Fv) and maximum fluorescence (Fm) to calculate the ratio of Fv/Fm. For determination of Pn, single individual leaves were placed in the leaf chamber with 400 μl L−1 CO2 at a light intensity of 800 μmol photon m−2 connected to the gas exchange analyzer (Li-Cor 6400, Li-Cor, Inc., Lincoln, NE, USA). Leaf Pn was then recorded until the reading became stable. Leaf EL was measured as an indicator of cell membrane stability using a conductivity meter (YSI Model 32, Yellow Spring, OH, USA) according to the procedure previously described by Blum & Ebercon[48].
Ionomic and nitrogen analysis
-
Leaf tissues were dried at 80 °C to a constant weight, ground into a fine powder, and then passed through a 40-mesh screen. For determination of total N, 0.25 g dry ash was placed in a 75 ml digestion tube and then 6 ml H2SO4 was added. After thoroughly mixing on a vortex stirrer, the digestion tube was preheated to 370 °C until all plant tissue was broken up. The digestion tube was removed from the digestion block and 4 ml 30% H2O2 was added. The tube was placed back on the digestion block and heated at 370 °C for 2 h. After removing the tube from the block and fumehood for 10 min, 15 ml deionized water was added. Sample digests can be analyzed for N on the vario Max C/N Analyzer (Elementar, Germany)[49]. The method of Miller[50] was used for determination of ionomics including P, K, Ca, Na, Mg, Mn, Fe, Cu, B, Al, and Zn. Leaf tissues were placed in a muffle furnace over 4 h at 500 °C and once at room temperature, ashed samples were removed from the furnace. Dry ash (0.25g) was dissolved in 10 ml of 1 N HCl solution. After digestion, ash digests were used for determination of mineral composition by using the Varian 730-ES Inductively Coupled Plasma Optical Emission Spectrometer (ICP-OES) (Agilent Technologies, USA).
Statistical analysis
-
The experiment was based on a split-plot design with temperature as the main plot and exogenous GABA application as the sub-plot. SAS (9.1, SAS Institute, Cary, NC, USA) was used to analyze treatment effects based on the general linear model procedure. Treatment differences were compared and tested using Fisher’s protected least significance test with P < 0.05.
This research was supported by the Center for Turfgrass Science at Rutgers University.
-
The authors declare that they have no conflict of interest.
- Copyright: © 2022 by the author(s). Published by Maximum Academic Press, Fayetteville, GA. This article is an open access article distributed under Creative Commons Attribution License (CC BY 4.0), visit https://creativecommons.org/licenses/by/4.0/.
-
About this article
Cite this article
Li Z, Burgess P, Peng Y, Huang B. 2022. Regulation of nutrient accumulation by γ-aminobutyric acid associated with GABA priming-enhanced heat tolerance in creeping bentgrass. Grass Research 2:5 doi: 10.48130/GR-2022-0005
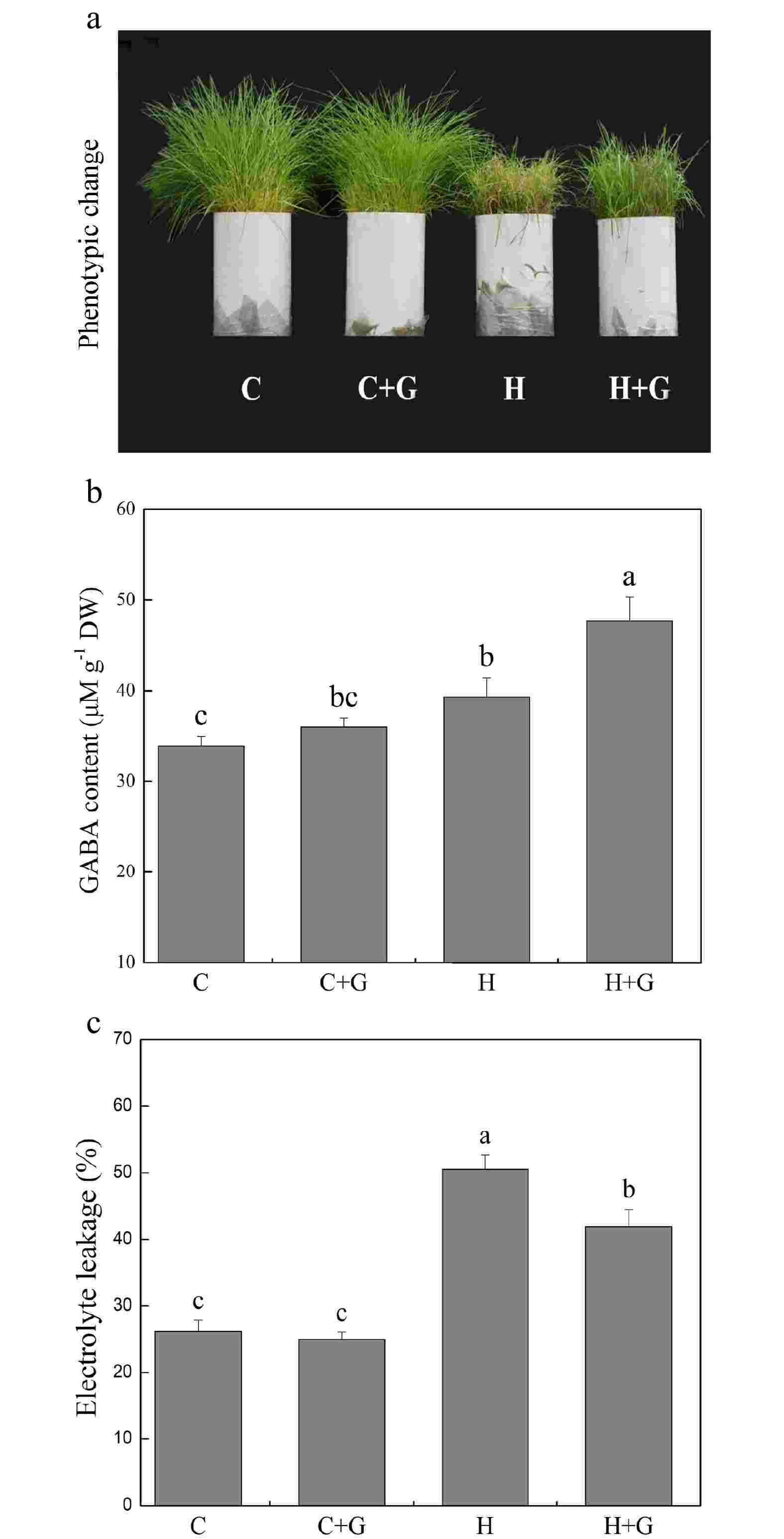