-
Chinese bayberry (Myrica rubra), a subtropical evergreen fruit tree belonging to the Myricaceae family, is predominantly found in southern China and other Asian countries[1]. The bayberry fruit, known for its distinctive taste and nutritional benefits, has gained significant economic value and consumer interest in recent years[2]. Chinese bayberries are particularly recognized for their high content of anthocyanins and various flavonoid substances, which serve as crucial secondary metabolites in plants. Anthocyanins play multifaceted roles in plant physiology, helping to modulate responses to environmental stressors such as ultraviolet radiation, high temperatures, and arid conditions. Additionally, these compounds are recognized for their capacity to help prevent a multitude of diseases[3,4]. Beyond their importance to plant health, these compounds have gained attention for their potential benefits to human health. Numerous studies have highlighted the capacity of anthocyanins to aid in preventing a wide array of diseases, making them a subject of keen interest in both nutritional and medical research[5,6].
The color transition in bayberry fruit, primarily driven by fluctuations in anthocyanin levels, serves as a clear indicator of the ripening process[7]. This visual transformation is underpinned by intricate pigment metabolic pathways and a sophisticated regulatory network. It has been established that the biosynthesis of anthocyanins involves a series of enzymatic reactions catalyzed by key enzymes including chalcone synthase (CHS), chalcone isomerase (CHI), flavanone 3-hydroxylase (F3H), dihydroflavonol 4-reductase (DFR), and anthocyanidin synthase (ANS)[8−10]. This process is further regulated by an array of transcription factors, notably R2R3-MYB, bHLH, and WDR, which exert control through diverse mechanisms[11,12]. Notably, genes including MYB1, MYB2, MYB10, MYB90, and MYB113 have been identified to exert pivotal control over the biosynthetic pathway of anthocyanins[13−15].
Light signals play a crucial role in plant growth and development, with a particularly significant impact during fruit maturation[16−18]. Previous studies have demonstrated that light exposure is essential for anthocyanin accumulation in various fruits. Both red and blue light have been shown to enhance the accumulation of anthocyanins in strawberries[19,20]. Combined multi-omics and bioinformatics analysis, it is revealed that red and white light exert distinct influences on the sugar metabolism within strawberry fruits, particularly affecting the accumulation of D-mannose-6-phosphate, sorbitol, and inositol[21]. In the context of tea plant anthocyanin synthesis, CsbHLH89 has been reported to bind to G-box elements within the promoters of CsCHS, CsFLS, and CsDFR, exerting a positive regulatory effect on anthocyanin synthesis[22].
Bagging, a common protective measure in horticulture, has been widely used to mitigate issues such as sunscald, disease occurrence, and chemical residues in fruits[23,24]. However, its effects on fruit pigmentation and quality, particularly in Chinese bayberries, have not been thoroughly investigated. Previous research has indicated that various bagging treatments can significantly influence the synthesis of pigments in Chinese bayberry fruits, with some treatments suppressing anthocyanin production[25,26]. The mechanisms underlying the accumulation of anthocyanins in Chinese bayberry fruits remain elusive. Therefore, these mechanisms are crucial for enhancing our knowledge of fruit development and improving cultivation techniques, and fruit quality.
The objective of this study is to investigate the effects of bagging and light manipulation on the pigmentation process in Chinese bayberry fruits, scrutinizing their contributions to fruit ripening, color development, and overall quality. Additionally, we identified and characterized key metabolites and genes involved in anthocyanin biosynthesis under varying light conditions using integrated transcriptomic and metabolomic analyses. Overall, this study advances our knowledge of the molecular basis of fruit coloration and also provides practical implications for improving fruit quality and cultivation practices in Chinese bayberries.
-
The 'Dong Kui' (Myrica rubra cv. DongKui Orient Pearl) served as the experimental material, with trees of identical age and growth conditions selected for the trial[27−29]. Cultivation was conducted under uniform management conditions. At veraison, some fruits were subjected to treatment with black no-transmitting bags (HZQ), ensuring that the microenvironments of the various bagging treatments were kept consistent. Bagging treatment was applied to adjacent fruits on the same branch, utilizing the black no-transmitting bags (HQ) and transmitting bags (WQ) during the green stage of the fruit's development, while a control group without bagging (CK) was also included. At maturity, fruits of uniform size were harvested. For each treatment, 15 fruits were collected to form a replicate, with three biological replicates employed for each sampling point. The samples collected for transcriptomic and metabolomic sequencing were rapidly frozen in liquid nitrogen and preserved at −80 °C to facilitate subsequent analyses.
Determination of physiological parameters
-
Fruit morphological and quality parameters were assessed immediately post-harvest. Equatorial and polar diameters of Chinese bayberry fruits were measured using a digital vernier caliper[30]. Individual fruit weights were measured using an analytical balance. The soluble solids content (SSC) was quantified using a PR-101 digital refractometer (ATAGO, Japan). The chroma of the fruits was assessed with a CR-10 handheld colorimeter (Konica Minolta Holdings, Inc., Japan), where the luminosity (L*), the red-green axis (a*), and the blue-yellow axis (b*) were recorded. Eleven measurements were conducted on five biological replicates, each consisting of 10 randomly selected fruits, to ensure statistical robustness and account for biological variability.
Determination of soluble sugar
-
The soluble sugar composition in Chinese bayberry fruits was determined through high-performance liquid chromatography (HPLC)[31]. Precisely 0.1 g pulverized fruit samples were extracted in 5 mL of an extraction solvent comprising a 50:50 ethanol-to-water ratio. Subsequently, the reaction mixture was subjected to heating in an 80 °C water bath for 30 min, followed by centrifugation at 4,500 × g for 15 min at 20 °C. This extraction process was repeated three times, and the supernatants were combined. The analysis of the samples was performed using HPLC (Agilent 1100, USA).
Determination of organic acid and vitamin C
-
Organic acids and vitamin C concentrations in Chinese bayberry fruits were quantified through HPLC[32]. A precisely weighed 1 g sample of the fruit tissue was homogenized in 5 mL of a potassium dihydrogen phosphate (KH2PO4) extraction solution. The homogenate underwent ultrasonic extraction at 40 kHz for 30 min at 4 °C, followed by centrifugation at 4,500 × g for 15 min at 4 °C. The supernatant was filtered through a 0.22 μm PTFE membrane before analysis. The subsequent analysis was performed using HPLC (Agilent 1100, USA), ensuring accurate determination of the analytes.
Determination of anthocyanin content
-
Bayberry fruit samples were pulverized in liquid nitrogen, and 0.1 g of the resulting powder was extracted with 1 mL of acidified ethanol (3% HCl in 80% ethanol, v/v)[33]. The mixture was homogenized thoroughly, sealed to prevent evaporation, and subjected to ultrasonic extraction for 30 min at 25 °C. Following centrifugation at 8,000 × g for 10 min at 20 °C, the supernatant was collected and adjusted to a final volume of 0.5 mL with the extraction solvent. The maximum absorption wavelength was determined by taking two separate 0.04 mL aliquots of the supernatant, each mixed with either 0.16 mL of potassium chloride buffer (0.025 mol/L, pH 1.0) or 0.16 mL of sodium acetate buffer (0.4 mol/L, pH 4.5). After 10 min of equilibration at room temperature, a full-spectrum scan (400−700 nm) was performed using a UV-Vis spectrophotometer, revealing a maximum absorption at 520 nm. For quantification, absorbance was measured at 520 and 700 nm for both pH conditions using fresh 40 μL aliquots of the supernatant mixed with their respective buffers. The difference in absorbance, ΔA, was calculated as the value obtained at pH 1.0 (A520−A700) minus that at pH 4.5 (A520−A700).
Determination of total phenol content
-
Total phenolic content was determined according to the Folin-Ciocalteu method[34]. Samples were pulverized in liquid nitrogen, and 0.100 ± 0.001 g of the resulting powder was extracted with 1 mL of acidified ethanol (3% HCl in 80% ethanol, v/v). The mixture was homogenized thoroughly, sealed to prevent evaporation, and subjected to ultrasonic extraction for 30 min at 25 °C. Following centrifugation at 8,000 × g for 10 min at 20 °C, the supernatant was collected and adjusted to a final volume of 0.5 mL with the extraction solvent. For the determination of the maximum absorption wavelength, the sample was again processed with liquid nitrogen, and approximately 0.1 g of the resulting powder was mixed with 0.5 mL of a 60% ethanol extraction solution. This mixture was ultrasonically extracted at a temperature of 60 °C for 30 min, followed by centrifugation at 12,000 revolutions per min for 10 min at a temperature of 25 °C. The supernatant was then collected for subsequent analysis. The assay was performed by mixing 10 μL of the extracted sample with 50 μL of Folin-Ciocalteu phenol reagent and 90 μL of distilled water. After 2 min at room temperature (22 ± 2 °C), 50 μL of the supernatant from the second extraction was added. The mixture was thoroughly vortexed and allowed to react at room temperature in darkness for 10 min. Absorbance was measured at 760 nm using a spectrophotometer.
Transcriptome analysis of bayberry fruit
-
Mature Chinese bayberry fruits were harvested at commercial ripeness from an orchard in Zhejiang Province, China. Fruits were carefully selected for uniformity in size and color and were free from visible defects or damage. The harvested samples were immediately flash-frozen in liquid nitrogen and stored at −80 °C for subsequent transcriptomic analysis. Each treatment consisted of five biological replicates, with each replicate comprising a pooled sample of fruits from ten independent plants. Total RNA was extracted using the CTAB method (MEGAN, Guangzhou, China)[29]. RNA integrity and purity were assessed using an Agilent 2100 Bioanalyzer (Agilent Technologies, Santa Clara, USA). RNA-Seq libraries were prepared using the VAHTS mRNA-Seq v2 Library Preparation Kit (Vazyme Biotech, Nanjing, China) according to the manufacturer's protocol. Sequencing was performed on an Illumina HiSeq X Ten platform (Illumina, San Diego, CA, USA) to generate paired-end reads of 150 bp in length.
Metabolomics analysis of bayberry fruit
-
Mature Chinese bayberry fruits were harvested at commercial ripeness and immediately flash-frozen in liquid nitrogen. The experimental design included five biological replicates per treatment, with each replicate consisting of a homogenized pool of fruits from ten independent plants to account for inter-plant variability. For non-targeted metabolite extraction, a methanol-based protocol was employed. Briefly, 100 mg of powdered fruit tissue was extracted with 1 mL of 80% methanol (v/v) by vortexing for 30 s, followed by ultrasonication for 30 min at 4 °C. The samples were then centrifuged at 12,000 × g for 15 min at 4 °C, and the supernatant was filtered through a 0.22 μm PTFE membrane[35]. Metabolite analysis was performed using an Agilent 1290 ultra-high-performance liquid chromatography (U-HPLC) system coupled to a high-resolution mass spectrometer.
Quantitative real-time PCR verification
-
Gene sequences were retrieved from the National Center for Biotechnology Information (NCBI) database. Quantitative real-time PCR (qRT-PCR) primers were designed using Primer Premier 5.0 software. The qRT-PCR reactions were performed using the TipGreen qPCR SuperMix fluorescent detection kit (Qingke, Beijing, China)[36]. Each 20 μL reaction mixture contained 10 μL of 2× TipGreen qPCR SuperMix, 0.4 μL each of forward and reverse primers (10 μM), 2 μL of cDNA template, and nuclease-free water to the final volume. The thermal cycling protocol consisted of an initial denaturation at 94 °C for 30 s, followed by 40 cycles of denaturation at 94 °C for 5 s and annealing/extension at 60 °C for 34 s. Gene expression was normalized to the pear β-actin gene, with primer sequences provided in the supplementary table. Relative gene expression levels were calculated using the 2−ΔΔCᴛ methods.
Statistics and reproducibility
-
Statistical analyses were conducted utilizing IBM SPSS, a renowned statistical software package, while graphical representations were crafted employing Prism 9.0, a specialized tool for data visualization. Statistical significance across all treatments was determined using Duncan's LSD analysis. Different letters indicate statistically significant differences in one-way ANOVA (p < 0.05).
-
The a*/b* values, a quantitative indicator of fruit red coloration, were employed to evaluate the effects of bagging treatments on Chinese bayberry pigmentation. Transmitting bags (WQ) did not significantly alter the a*/b* ratio compared to unbagged controls, although fruit luster was notably enhanced. In contrast, black no-transmitting bags (HQ) significantly reduced pigment deposition in mature fruits, resulting in a marked increase in a*/b* values and superior luster compared to other treatments, also synchronously-decreased the total phenols (60.1%), anthocyanin (90.8%) content (Fig. 1).
Figure 1.
(a), (b) Effect of fruit color, (c) luminosity, and (d) anthocyanin in mature Chinese bayberry after treatment with different light-transmitting bags. Bars represent the mean and standard error of three independently transformed biological replicates (n = 3). Different letters indicate statistically significant difference in one-way ANOVA analysis (p < 0.05). HQ: Blank-no-transmitting bag treatment; WQ: white-light treatment.
To elucidate the influence of bagging and light exposure on commercial fruit attributes, we assessed multiple quality parameters of mature fruits. Bagging during early fruit development significantly impacted fruit quality, characterized by decreased total sugars (36.14%), sucrose (55.3%), glucose (59.5%), and fructose levels (37.5%), coupled with increased total acidity (71.1%), malic acid (36.6%), and oxalic acid concentrations (46.5%) (Supplementary Fig. S1). Notably, bagging during veraison did not significantly alter total sugar or acid content. The HQ treatment marginally reduced sugar-acid content compared to the WQ treatment. Remarkably, all bagging treatments substantially decreased fruit vitamin C (93.0%) content, with HQ treatment exerting the most pronounced effect. These findings suggest that the pre-veraison period is critical for sugar and acid accumulation, while the veraison-to-maturity phase is crucial for sugar-acid interconversion and vitamin C synthesis (Supplementary Fig. S1). We found that early-stage bagging severely impedes photosynthetic product accumulation, whereas bagging from veraison to maturity primarily affects vitamin C synthesis and sugar-acid metabolism.
Light-induced transcriptomic changes in Chinese bayberry fruit
-
To elucidate the light-regulated differences in pigment composition within Chinese bayberry fruit, we conducted comprehensive transcriptomic and metabolomic analyses on samples harvested at maturity from the HQ and WQ treatments. Principal Component Analysis (PCA) was employed to synthesize and interpret the transcriptomic data derived from these distinct bagging treatments (Fig. 2c−e). The first principal component (PC1) accounted for a significant proportion of the variance in transcriptomic datasets, while the second principal component (PC2) elucidated additional variability within the data (Fig. 2a). Transcriptomic profiling revealed 14,851 genes co-expressed across HQ and WQ treatments demonstrated differential expression of 938 genes, with 335 genes upregulated and 603 genes downregulated in the opaque treatment (Fig. 2b). KEGG pathways enrichment analysis identified that the differential gene expression attributed to light exposure was predominantly concentrated within the phenylpropanoid biosynthesis pathway, the MAPK signaling pathway, and the flavonoid metabolic pathway.
Figure 2.
Transcriptome analysis of Chinese bayberry bagged with two light-transmitting bags. (a) PCA analysis, (b) volcano plot (c) heatmap clustering, (d) KEGG analysis, and (e) Venn analysis the treatments with black no-transmitting bags (HQ) and transmitting bags during (WQ).
Metabolism analysis of light-transmitting bags treatment related to anthocyanin synthesis
-
Metabolomic sequencing data revealed significant enrichment of differential metabolites in pathways associated with signal transduction, phenylpropanoid biosynthesis, and carbon metabolism (Fig. 3). We characterized six principal classes of metabolites associated with anthocyanin biosynthesis from the pulp of 'Dongkui' Chinese bayberries, including proanthocyanidins, anthocyanins, phenolic acids, flavonoids, flavonol glycosides, and coumarins through advanced metabolomic techniques. Our analysis identified a total of 44 differentially expressed metabolites implicated in anthocyanin synthesis. Notably, in the WQ treatment, we observed a significant elevation in the levels of specific compounds such as proanthocyanidins (B2, B3, B4), pelargonidin glucoside, and paeonidin glucoside. These compounds are likely the primary contributors to the characteristic red pigmentation observed in Chinese bayberry fruit under light-transmitting bag conditions (Fig. 4). This metabolomic profile provides crucial insights into the biochemical mechanisms underlying fruit coloration in response to light exposure. The elevated levels of proanthocyanidins and specific anthocyanins in the WQ treatment suggest that light transmission plays a key role in modulating the biosynthesis and accumulation of these pigment-related compounds.
Figure 3.
Metabolism analysis of Chinese bayberry bagged with two light-transmitting bags. (a) PCA analysis, (b) volcano plot, (c) KEGG analysis, and (d) heatmap clustering analysis the treatments with black no-transmitting bags (HQ) and transmitting bags during (WQ).
Figure 4.
Cyanidin profiles in Chinese bayberry fruit treated with bags of varying light transmittance. (a) A visual heat map of cyanidins in Chinese bayberry fruit. Metabolite content is represented by peak area values. (b) Differences in cyanidin content in Chinese bayberry fruit subjected to different bagging treatments.
Effects of light-transmitting bags treatment on the expression of anthocyanin-related genes
-
Transcriptomic profiling identified all genes associated with the biosynthesis and glycosylation of anthocyanins. KEGG analysis revealed that within the HQ treatment, there was a significant upregulation in the expression of genes such as chalcone synthase (CHS) and flavonol synthase (F3'H), while the expression of the leucoanthocyanidin dioxygenase (LDOX) gene was noticeably reduced. These expression patterns aligned with the observed decrease in total anthocyanin content in the HQ treatment (Fig. 5). These findings suggest that flavonoid synthesis was not suppressed under reduced light conditions in the WQ treatment. The elevated expression of LDOX in WQ may be primarily responsible for maintaining the coloration of Chinese bayberry fruit under these conditions. Pelargonidin was identified as a significant pigmented anthocyanin within the fruit, likely contributing to the observed coloration patterns. To validate the RNA-Seq data, we conducted qRT-PCR analysis (Fig. 6) providing robust support for our transcriptomic observations. These transcriptomic insights, coupled with our metabolomic findings, offer a comprehensive view of the molecular mechanisms underlying anthocyanin biosynthesis and accumulation in Chinese bayberry fruit under different light conditions.
Figure 5.
Transcriptome analysis of the expression patterns of anthocyanin-related genes. HQ: black no-transmitting bags, WQ: transmitting bagsduring. Green to red color indicate the gene expression increasing abundance.
Figure 6.
qRT-PCR analysis of the expression levels of DEGs identified from RNA-Seq. (a) HY5: Transcription factor HY5; (b) F3'H: flavonol synthase; (c) ERF098: Ethylene-responsive transcription factor ERF098; (d) MYB/DF1: MYB-like protein; (e) G-1,3-β-glucosidase: Glucan endo-1,3-beta-glucosidase; (f) CYP82D47: Cytochrome P450 CYP82D47; (g) CYP73A100: Cytochrome P450 CYP73A100; (h) PGIP: Polygalacturonase inhibitor. Error bars indicate standard error from three biological replicates (n = 3). Asterisks indicate a statistically significant difference in one-way ANOVA analysis (* p < 0.05, ** p < 0.01, *** p < 0.001).
An integrative analysis was conducted on the eight most significant differential metabolites identified, revealing a cohort of ten transcription factors and genes that participate in the light-mediated regulation of anthocyanin biosynthesis. Notably, the transcription factors HY5 and ERF098 exhibited positive correlations with both the levels of anthocyanins and the expression of genes involved in anthocyanin biosynthesis, whereas the transcription factor DF1 demonstrated an inverse relationship with these parameters (Fig. 7). The transcription factor HY5 emerged as a central regulatory element within the regulatory network. These study suggest that the Chinese bayberry HY5 proteins may have undergone functional diversification. The unique phylogenetic position of the Chinese bayberry HY5 protein, combined with its central role in the regulatory network of anthocyanin biosynthesis, highlights its potential significance in the species-specific regulation of fruit pigmentation (Supplementary Fig. S2). These insights contribute significantly to the understanding of the molecular underpinnings of light-regulated pigmentation in Chinese bayberry fruit.
Figure 7.
The correlation between light-regulated anthocyanins through integrated transcriptome and metabolomics data. Pentagons represent metabolites, triangles denote genes, circles indicate microorganisms, and proteins are depicted as rectangles. Microorganisms can be classified at various taxonomic levels, such as the genus level. If metabolites have specific names, these names are used in place of identifiers. Red lines signify positive correlations, while green lines indicate negative correlations; the thicker the line, the stronger the correlation.
-
Light signals play a pivotal role in the growth, development, and quality attributes of fruit[37,38]. However, the specific influence of light on the development of Chinese bayberry fruit has remained elusive. Bagging, a prevalent horticultural technique that controls light exposure, has not been previously explored for its impact on the ripening process of Chinese bayberries. This study employed a range of bagging treatments with varying light transmittance to examine the effects of light quality on the maturation of Chinese bayberries at both the metabolic and molecular levels. Our study revealed that black non-transmitting bag treatments significantly diminished the quality of Chinese bayberry fruit. Previous studies have shown that blue light treatment can significantly increase the content of sucrose, fructose, and glucose during storage[39]. Additionally, elevated levels of glucose, fructose, and sucrose were observed in developing tomato fruits subjected to RB31 light treatment[40]. Our findings indicate that a deficiency in light exposure directly leads to a decline in fruit quality, characterized by a reduction in sugar, and acid content and an increase in citric acid concentration (Supplementary Fig. S1). This suggests that light is essential for the synthesis or transportation of photosynthetic products. Moreover, this trend is consistent with the synthesis process of anthocyanins, indicating a potentially strong correlation.
Light exposure is essential for anthocyanin accumulation in various fruits[18,41]. Anthocyanins, natural pigments that accumulate in plants grown under light conditions, are subject to inhibition in the absence of light by the ubiquitin ligase complex COP1/SPA, which is composed of the photomorphogenesis gene/suppressor PHYA-105[42−44]. Our research found that the application of black non-transmitting bagging (HQ) during the early fruit stage led to a suppression of color development, manifesting as a lack of pigmentation. Conversely, the application of black no-transmitting bags treatment (HQ) during the veraison did not impede the fruit's coloration, suggesting that pigment accumulation material in the fruit occurs before this phase. The transformation from colorless precursors to pigmented anthocyanins during veraison appears to be independent of light exposure. Hence, the pattern of color accumulation throughout the development of Chinese bayberry fruit may be associated with light-induced anthocyanin synthesis[16,39].
For example, Jiang et al.[38] reported that the biosynthesis of anthocyanins in eggplant is entirely dependent on light, with no accumulation occurring in darkness. In our research, we observed that light treatment significantly influences the accumulation of anthocyanins within the fruit by modulating the expression of leucoanthocyanidin dioxygenase (LDOX). However, dark treatment during the phase of anthocyanin accumulation does not alter the final anthocyanin content, suggesting that the regulatory effect of light on anthocyanin accumulation in Chinese bayberry fruit is not sustained but rather occurs at specific developmental stages. An integrative analysis of the transcriptome and metabolome revealed that the transcription factor HY5, positioned centrally within the regulatory network, likely serves as a molecular switch in the transduction of light signals, engaging with downstream target proteins.
The anthocyanin synthesis pathway is regulated in multiple ways. Specifically, the blue light receptors CRY1/CRY2 have been found to interact with COP1[44−46], modulating the binding of transcription factors HY5 and MYB1 to the promoters of downstream anthocyanin biosynthetic genes (CHS and DFR), thus influencing the anthocyanin synthesis pathway within the fruit. Furthermore, the light signal transduction factor CsHY5 is capable of binding to the promoter of CsbHLH89, indirectly facilitating the accumulation of anthocyanins[22]. In the case of carotenoid synthesis, HY5 has been identified to negatively regulate the accumulation of carotenoids under light conditions, as evidenced by the orange color of carrots grown underground and their green appearance when exposed to light[47]. Consequently, HY5, functioning as a light signal receptor, initiates a cascade of signal transduction pathways that influence pigment biosynthesis and distribution, although with notable variations in the mechanisms of material accumulation and regulation among different species.
HY5, a basic leucine zipper (bZIP) transcription factor, plays a pivotal role in photomorphogenesis and the regulation of plant growth and development. It is known to interact with COP1 through BRLZ and WD40 domains of SmHY5[48−50]. In our study, bagging experiments with varying light transmittance revealed that the application of black bags, which prevented light exposure to the fruit, resulted in a near-complete suppression of HY5 gene expression. The HY5 transcription factor is implicated in the regulation of anthocyanin accumulation within the fruit through multiple pathways. In grapevines, VvMYBA1 and VvMYB24 have been identified as critical regulators of fruit coloration. Research has shown that MdHY5 upregulates the expression of MdMYB1 by binding to the G-box element in its promoter, consequently enhancing anthocyanin production in apples[45]. Additionally, VvHY5 has been demonstrated to bind to the promoter of VvMYB24, activating its transcription and thereby influencing the biosynthesis of anthocyanins in grapes[51]. Collectively, these findings suggest that HY5 functions as a key component in the light signaling pathway, acting as a molecular switch to directly or indirectly modulate the synthesis of anthocyanins. This mechanism highlights the intricate relationship between light perception and fruit pigmentation, underscoring the importance of light-mediated transcriptional regulation in fruit development and quality.
-
This comprehensive study reveals the intricate mechanisms underlying light-regulated anthocyanin biosynthesis in Chinese bayberry fruit. We discovered the critical role of light exposure in fruit quality development and pigmentation through integrated transcriptomic and metabolomic analyses. Notably, black no-transmitting bagging during early fruit stages significantly reduced pigment deposition, sugar accumulation, and overall fruit quality. Our findings demonstrated that the LDOX gene is pivotal in anthocyanin biosynthesis, with pelargonidin emerging as the predominant anthocyanin under light-exposed conditions. Furthermore, we identified the transcription factor HY5 as a central regulator in the light-mediated anthocyanin accumulation pathway. These insights provide a groundbreaking perspective on the intricate relationship between light exposure, bagging treatments, and fruit quality development during ripening. Additionally, this research paves the way for innovative breeding strategies and advanced horticultural practices, potentially revolutionizing fruit production across multiple crops.
The work was supported by the 'Lingyan' R&D in Zhejiang (2023C02031), the special breeding program for new varieties in Zhejiang (2021C02066-2), the Project of Xianghu Laboratory (Grant No. 2023C1S02002) and Technology Planning Projects of Zhejiang Province (2024SSYS0100).
-
The authors confirm contribution to the paper as follows: study conception and design: Yang H; data collection: Sun L, Qi Y; analysis and interpretation of results: Li Z, Lei K; draft manuscript preparation: Cheng F, Lei Y; program management: Wu Y, Ying Z; editing: Ahmed T, Yu Z; funding management: Qi X, Zhang S. All authors reviewed the results and approved the final version of the manuscript.
-
All data generated or analyzed during this study are included in this published article and its supplementary information files.
-
The authors declare that they have no conflict of interest.
-
# Authors contributed equally: Han Yang, Li Sun
- Supplementary Fig. S1 Effect of Chinese bayberry bagged with two light-transmitting bags on the 555 Total sugar (a), total flavone (b), Total acid (c), Vitamin C (d), sucrose, glucose, and fructose content (e), and malic acid, citric acid, oxalate content (f). Error bar indicate standard error from three biological replicates (n = 3). HQ: Blank-light treatment; WQ: white-light treatment; Different letters indicate statistically significant difference in one-way ANOVA analysis (p < 0.05).
- Supplementary Fig. S2 Phylogenetic tree of HY5 proteins from different plants. The tree was 561 generated using the ML method with MEGA6.0, and the bootstrap value was set as 1000. Oryza sativa Japonica Group transcription factor HY5 (LOC4327123); Ziziphus jujuba transcription factor HY5-like (LOC107433384); Glycine soja transcription factor HY5-like (LOC114406314); Prunus persica transcription factor HY5-like (LOC18792251); Mangifera indica transcription factor HY5-like (LOC123221145); Pistacia vera transcription factor HY5-like (LOC116144536); Prunus avium transcription factor HY5-like (LOC110755375); Vitis vinifera transcription factor HY5-like (LOC100261472); Pyrus x bretschneideri transcription factor HY5-like (LOC103944715); Malus domestica transcription factor HY5-like (NM_001293960.1); Arabidopsis thaliana HY5-homolog (NM_001084700.2); Corylus avellana transcription factor HY5-like (LOC132165499); Quercus suber transcription factor HY5-like (LOC112020306); Alnus glutinosa transcription factor HY5-like (LOC133858536); Carya illinoinensis transcription factor HY5-like (LOC122316597); Juglans regia transcription factor HY5-like (LOC109005672).
- Copyright: © 2025 by the author(s). Published by Maximum Academic Press, Fayetteville, GA. This article is an open access article distributed under Creative Commons Attribution License (CC BY 4.0), visit https://creativecommons.org/licenses/by/4.0/.
-
About this article
Cite this article
Yang H, Sun L, Qi Y, Li Z, Lei K, et al. 2025. Integrated transcriptomic and metabolomic analysis reveals light-induced modulation of anthocyanin biosynthesis in Chinese bayberry (Myrica rubra). Fruit Research 5: e015 doi: 10.48130/frures-0025-0004
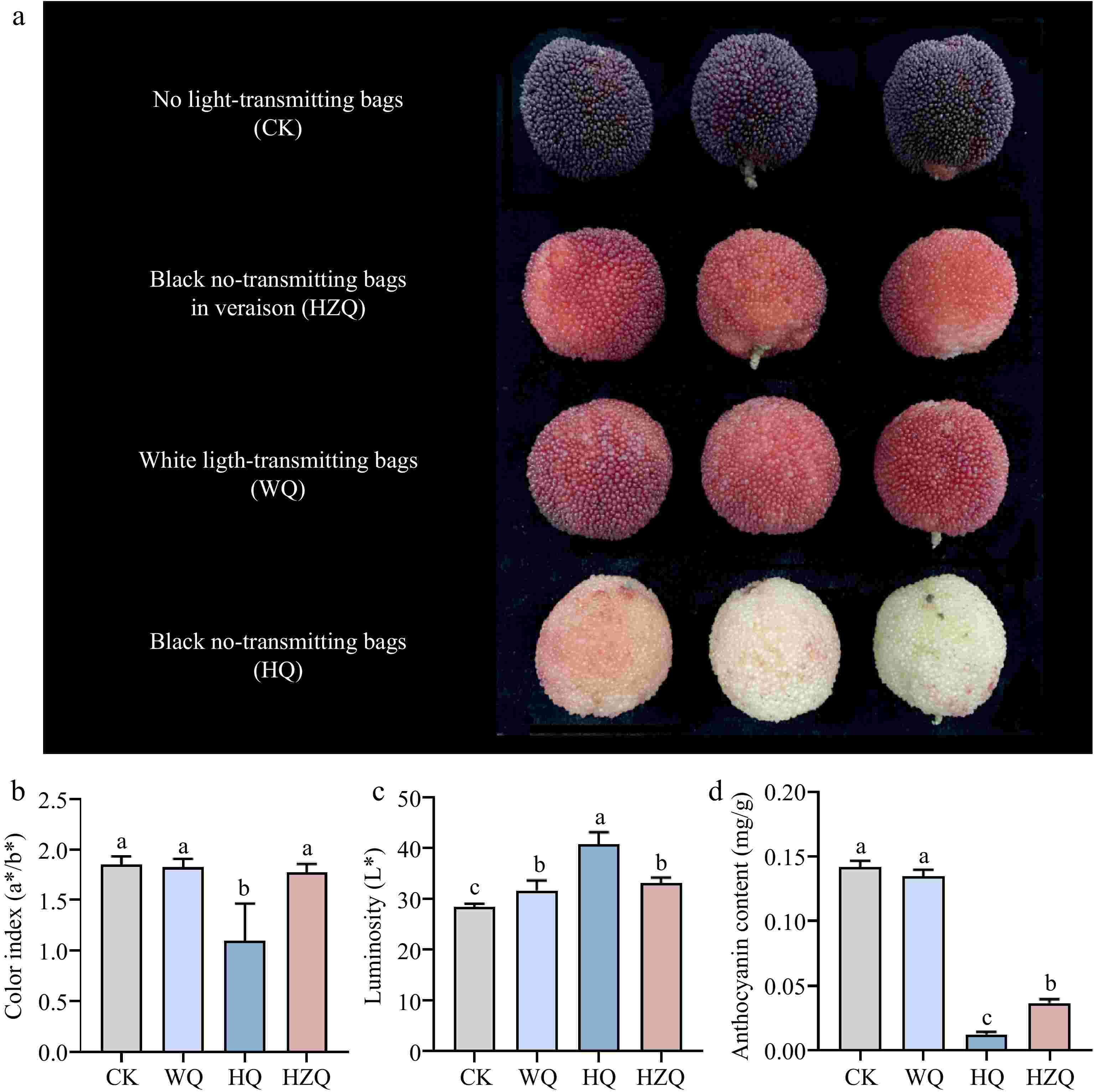