-
Zero-carbon renewable clean energy is a critical pathway for China to achieve its carbon peaking and carbon neutrality goals, as well as a new driving force for promoting high-quality economic and social development[1]. Hydrogen, as a highly promising zero-carbon energy carrier[2,3], can be produced through clean energy sources such as solar energy and renewable electricity[4−6]. The large-scale utilization of hydrogen in gas turbines is of great significance for realizing the dual carbon targets. However, hydrogen's highly reactive chemical properties, combined with its fast flame propagation speed, make it prone to issues such as flashback and thermoacoustic instabilities, leading to combustion instability in gas turbine systems[7,8]. Methane, the primary component of natural gas, is currently a significant fuel for gas turbines. Co-firing hydrogen with methane can partially replace methane and serve as a key approach to modulate fuel reactivity and facilitate hydrogen utilization. Therefore, it is essential to gain a deeper understanding of the ignition characteristics under both pure hydrogen combustion, and hydrogen-methane co-firing conditions.
Based on shock tubes and rapid compression machines, experimental and modeling studies on the ignition characteristics of hydrogen/methane mixtures have been conducted. These studies primarily focus on the effects of factors such as temperature, pressure, equivalence ratio, and blending ratio on ignition delay times (IDTs). Petersen et al.[9] investigated the ignition characteristics of hydrogen/methane mixtures with methane blending ratios of 20% and 40%. The results indicated that an increase in the methane proportion significantly lengthened the IDTs, while the co-firing of methane did not alter the overall activation energy. Herzler & Naumann[10] measured the IDTs of hydrogen/methane/ethane mixtures with hydrogen content ranging from 0% to 100% under conditions of 900–1,800 K and 1.0–16.0 atm using a shock tube. They also predicted the IDTs using mechanisms such as GRI Mech 3.0[11]. A comparison revealed that mechanisms like GRI Mech 3.0[11] struggled to accurately capture the reduction in activation energy at low temperatures in systems with high hydrogen content. Zhang et al.[12,13] investigated the ignition characteristics of hydrogen/methane mixtures in a shock tube at pressures ranging from 5 to 20 atm, with methane proportions varying from 0% to 100%. The results similarly demonstrated that the ignition temperature of the reaction system increased significantly with the rise in methane proportion. These measurements were used to validate models such as the NUI Galway Mech[11], and USC Mech 2.0[14]. However, the performance of different models in predicting IDTs varied considerably, especially under pure hydrogen conditions. It was thus observed that the current models exhibit significant discrepancies in their predictions for different hydrogen/methane blending ratios, failing to achieve accurate predictions across a broad range of conditions. Donohoe et al.[15] studied the ignition delay times of hydrogen/methane mixtures over various temperatures and pressures using shock tubes, and rapid compression machines. Panigrahy et al.[16] focused on comparing the reaction mechanisms of hydrogen, methane, and hydrogen-methane mixtures across different temperature ranges, explaining the intrinsic mechanism behind the long ignition delay time of hydrogen compared to methane and hydrogen-methane mixtures in the low-temperature regime.
In addition, CO2 is widely used in combustion processes such as oxy-fuel combustion and exhaust gas recirculation (EGR). The next generation of gas turbines will adopt hydrogen-rich blending, pure oxygen combustion, and Allam cycle technology to achieve flexible fuel use, low emissions, and high efficiency. In oxy-fuel combustion with carbon-containing fuels, the products are a steam-CO2 mixture, which allows for high-concentration CO2 capture through a simple steam condensation and separation process, enabling near-zero CO2 emissions. Moreover, as the working fluid and fuel are nitrogen-free, this fundamentally eliminates the issue of NOx emissions. Karimi et al.[17] studied the IDTs of syngas in CO2 and Ar atmospheres. Their kinetic analysis suggested that CO2 competes with the production of OH radicals. However, contrary to expectations, the experimental results did not reveal any significant difference in IDTs between the CO2 and Ar atmospheres. The authors attributed this to a combined kinetic effect, but such an explanation is unconvincing. Shao et al.[18] investigated the differences in IDTs of H2 and CH4 in 85% CO2 atmospheres. The model showed good agreement with experimental data at high pressures. However, the sensitivity analysis did not identify any reactions involving CO2, which contradicts the kinetic analysis by Karimi et al.[17]. Additionally, it is unfortunate that Shao et al.'s study did not compare other dilution atmospheres, making it impossible to determine whether CO2 had any influence on the experimental results. Subsequently, Harman-Thomas et al.[19] investigated the IDTs of H2 under varying CO2/N2 concentrations. Their findings revealed a significant difference in IDTs between H2 in CO2 and N2 diluted atmospheres. Kinetic analysis demonstrated that CO2 participates in competition for H radicals, further influencing the ignition delay time of the fuel. In summary, it can be observed that the current research has relatively few studies addressing the impact of CO2 on the IDTs of CH4/H2 fuel mixtures.
In this study, three representative fuel compositions with potential for gas turbine applications were selected for hydrogen/methane mixtures: 100% H2, 50% H2/50% CH4, and 30% H2/70% CH4. Under conditions using CO2 and Ar as diluent gases, the ignition characteristics were investigated using a shock tube at 950–2,100 K, 1.2–5.0 atm, and an equivalence ratio (φ) of 0.5, with IDTs measured under various operating conditions. A kinetic model for H2/CH4 combustion under different diluent atmospheres (Ar and CO2) was constructed and validated using the experimental data. Based on this model, reaction rate analysis and sensitivity analysis were conducted to explore the kinetic mechanisms influencing ignition characteristics under different pressures and blending ratios. This provides foundational data support for the large-scale, stable combustion of hydrogen in gas turbine applications.
-
The experiments in this study were conducted using the shock tube experimental platform at Shanghai Jiao Tong University (Shanghai, China). As shown in (Fig. 1), the platform primarily consists of four components: the shock tube body, the exhaust system, the gas mixing and delivery system, and the signal acquisition system. The shock tube body is constructed from stainless steel, with an inner diameter of 92 mm. The driver section is 3.5 m in length, while the driven section extends 5.5 m. The diaphragm separating the two sections is ruptured using the cross-blade method. The exhaust system consists of three stages: an oil pump, a roots pump, and a molecular pump. The gas mixing and delivery system primarily includes a premix tank and gas cylinders, with the mixture prepared using Dalton's partial pressure method. The gas mixture is left to stand for 12 h to ensure thorough premixing. The signal acquisition system comprises five dynamic pressure sensors located along the driven section, a signal conditioning instrument, a photomultiplier tube (PMT), and a computer for data processing. During the experiment, the shock tube was evacuated stepwise using an oil pump, a roots pump, and a molecular pump. Once the pressure dropped below 3 Pa, the driven and driver sections were filled with gas. He was used as the driver gas. When the pressure difference between the driver and driven sections reached the diaphragm rupture pressure, the polyester diaphragm ruptured, generating an incident shock wave. The pressure jump caused by the incident shock was monitored by dynamic pressure sensors, with the signals converted into voltage through a charge amplifier and signal conditioner. The velocity of the incident shock wave was calculated based on the arrival times at each pressure sensor and the distances between the sensors. The velocity of the reflected shock wave at the tube end was then extrapolated using a linear relationship. The OH emission signal generated during fuel ignition was synchronously recorded by PMT after signal conditioning. The reaction temperature (T5) and pressure (P5) behind the reflected shock wave were calculated using MATLAB, based on the experimental conditions and shock wave properties.
The definition of ignition delay time is illustrated in Fig. 2. To avoid difficulties in determining IDTs under low-temperature conditions, where weak ignition and minimal pressure rise may make IDTs determination unclear, the ignition delay time is uniformly defined as the intersection point between the extrapolated line at the maximum slope of the OH signal and the horizontal baseline. It should be noted that the non-ideal boundary layer effects measured by the change in pressure (dP/dt) behind the reflected shock wave were determined to be less than 4%/ms. The experimental measurement error for IDTs is estimated to be 20%. The fuels used in this study were 100% H2, 50% H2/50% CH4, and 30% H2/70% CH4. The mixtures were diluted with 90% Ar, except for case 7, which used 90% CO2 as the diluent. The equivalence ratio for all cases was set at 0.5. The specific cases are listed in Table 1.
Table 1. Experimental conditions for the ignition delay time measurements.
No. Equivalence ratio XH2/(XH2 + XCH4) H2 (%) CH4 (%) O2 (%) Dilution gas (%) Pressure (atm) 1 0.50 1.00 5.00 0.00 5.00 90.00 Ar 1.20 2 0.50 1.00 5.00 0.00 5.00 90.00 Ar 5.00 3 0.50 0.50 1.43 1.43 7.14 90.00 Ar 1.20 4 0.50 0.50 1.43 1.43 7.14 90.00 Ar 5.00 5 0.50 0.30 0.73 1.71 7.56 90.00 Ar 1.20 6 0.50 0.30 0.73 1.71 7.56 90.00 Ar 5.00 7 0.50 0.50 1.43 1.43 7.14 90.00 CO2 1.20 Reaction kinetic model
-
Based on the previously developed C0-C4 core combustion reaction mechanism from previous research[20,21], a combustion reaction mechanism for hydrogen and methane (hereafter referred to as SJTU Mech) was developed. The mechanism consists of 43 species and 388 reactions. The hydrogen sub-mechanism is primarily derived from the hydrogen model developed by Hashemi et al. in 2015[22]. The reaction H+O2
O+OH adopts the reaction rate constants determined by Yu et al.[23] based on shock tube measurements. The methane sub-mechanism is mainly adopted from the methane model developed by Jin et al.[24], which includes methane unimolecular reactions, hydrogen abstraction reactions, and subsequent reactions of CH3. The rate constants for the reactions CH3+H(+M)⇌ CH4(+M) and CH4+H⇌ CH3+H2 are taken from the shock tube measurements by Wang et al.[25] and Srinivasan et al.[26], respectively. Sutherland et al.[27] measured the rate constants for the reaction CH4+H⇌ CH3+H2 using shock tube laser photolysis coupled with H-atom absorption spectroscopy. The present model adopts their measured results. The rate constants for hydrogen abstraction reactions of methane with O2, CH3, and O are taken from GRI Mech 3.0[28] and the evaluations by Baulch et al.[29]. The generated CH3 can undergo recombination reactions with species such as H and CH3, as well as oxidation reactions with O2, O, and HO2, leading to the formation of formaldehyde. The model also includes the rapid dissociation reaction of HCO proposed by Labbe et al. in 2016[30]. The HCO generated from formaldehyde hydrogen abstraction reactions is highly energetic; part of the HCO de-excites to form stable HCO, while another portion may dissociate rapidly without de-excitation to form CO and H, known as the rapid dissociation of HCO. This reaction facilitates the direct and efficient production of H atoms in formaldehyde hydrogen abstraction, thereby enhancing the reactivity of the combustion system. As a crucial component in technologies such as oxy-fuel combustion, biogas, and flue gas recirculation, CO2 is an important factor. In this model, the reaction rate constants for the reaction CO2+H⇌ CO+OH were re-evaluated, utilizing the rate constants from the model developed by Zhang et al.[12]. Additionally, this study compares the performance of various core mechanisms, including NUI Galway Mech[11], USC Mech 2.0[14], and GRI Mech 3.0[28], in predicting IDTs. The simulation of IDTs was conducted using the Closed Homogeneous Batched Reactor module of Chemkin-Pro[31].⇌ H+O2⇌O+OH (R1) CH4+H⇌CH3+H2 (R2) CH3+H(+M)⇌CH4(+M) (R3) -
Figure 3 presents a comparison of experimental measurements and model predictions of IDTs for 100% H2, 50% H2/50%CH4, and 30% H2/70% CH4 under pressures of 1.2 and 5.0 atm. There is a strong linear relationship between IDTs and 1/T when the pressure is 1.2 atm. As the proportion of methane increases, the IDTs curves shift progressively toward higher temperatures. Notably, when the methane content reaches 70%, the ignition temperature significantly increases, and the IDTs also rise sharply. This indicates that an increase in methane proportion reduces the reactivity of the fuel mixture, requiring higher temperatures for ignition, thereby illustrating the ability of methane to flexibly modulate the reactivity of the mixture over a wide range. Furthermore, it can be observed from the figure that at 1.2 atm, the differences among the various models are minimal, all accurately predicting the experimental results.
Figure 3.
Comparison of experimental (dots) and simulated (lines) results of IDTs for 100% H2, 50% H2/50% CH4, and 30% H2/70% CH4 at 1.2 and 5.0 atm.
As the pressure increases, the IDTs curves shift toward the lower temperature region. At a pressure of 5.0 atm, there is a strong linear relationship between IDTs and 1/T under the conditions of 50% CH4, and 70% CH4. However, under direct hydrogen combustion conditions, the IDTs curves exhibit significant differences across various temperature ranges. Specifically, IDTs remain relatively low within the temperature range of 1,040–1,250 K, while they increase sharply below 1,040 K, reflecting the highly reactive nature of hydrogen combustion. Under the 5.0 atm condition, different models yield consistent predictions for IDTs in the high-temperature region, but discrepancies arise in the low-temperature region, particularly under direct hydrogen combustion, where the deviations are most pronounced. SJTU Mech demonstrates good predictive performance for experimental results across different conditions. NUI Galway Mech and USC Mech 2.0 are well-developed, thus exhibiting reasonable prediction errors for hydrogen. In contrast, GRI Mech 3.0, primarily developed for methane combustion, shows larger prediction errors under these conditions; however, the predictions improve as the proportion of methane increases. A comprehensive comparison of IDTs results for various hydrogen-to-methane ratios indicates that the incorporation of methane allows for flexible adjustment of the reactivity and ignition characteristics of the H2/CH4 mixture over a wide range.
To reveal the kinetic mechanisms at different blending ratios, reaction pathway analyses were conducted based on SJTU Mech for two blending ratios: 100% H2 and 30% H2/70% CH4 under conditions of 1,100 K and 5.0 atm, as shown in Fig. 4. For 100% H2, more than 75% of the hydrogen is directly converted into the stable product H2O through hydrogen abstraction reactions with OH. A smaller portion of hydrogen is transformed into radicals such as H and OH through oxidation and hydrogen abstraction reactions. Most of the H radicals are further converted into OH radicals, which are ultimately converted into H2O. It can be observed that hydrogen can directly generate reactive hydrogen atoms through a few reaction sequences, which then undergo chain branching reactions. This explains why hydrogen has high reactivity, ignites easily, and results in shorter IDTs.
For the 30% H2/70% CH4 mixture, CH4 is primarily consumed through hydrogen abstraction reactions with various radicals such as OH, O, and H, leading to the formation of CH3. Under lean combustion conditions, hydrogen abstraction by OH and O radicals accounts for 85% of methane consumption. CH3 predominantly undergoes oxidation reactions to further convert into CH2O, with some forming CH3O, which almost entirely converts back into CH2O. CH2O then undergoes hydrogen abstraction to form HCO, which is gradually converted into CO and CO2. Thus, CH3 and CH2O are the key intermediates in methane combustion.
Comparing the two blending ratios reveals that, in the methane-dominated 30% H2/70% CH4 reaction system, the oxidation process led by CH3 is influenced by hydrogen. Hydrogen provides an abundant supply of OH and H radicals, which accelerates the conversion of CH4 to CH3 and further to CO2. In contrast, in the hydrogen-dominated system, CH3 does not play a role, and the reaction process is much simpler compared to the system involving methane. The addition of methane alters the reaction pathways, thereby controlling the reaction rate of the mixed fuel system, ensuring stable combustion of hydrogen.
To investigate the key reactions controlling ignition at different blending ratios, IDTs sensitivity analyses were conducted for 100% H2, 50% H2/50% CH4, and 30% H2/70% CH4 under conditions of 1,100 K and 5.0 atm. The sensitivity coefficient is defined as follows[12,13]:
S=τ(2ki)−τ(0.5ki)1.5τ(ki) where, S represents the sensitivity coefficient, τ is the ignition delay time, and ki is the rate constant of reaction i.
The sensitivity analysis results are shown in Fig. 5. It can be observed that for mixtures with different blending ratios, IDTs are consistently influenced by the reaction R1 H+O2
O+OH, which is the most critical chain-branching reaction in the system and significantly promotes ignition, thus exhibiting negative sensitivity. In contrast, reactions with positive sensitivity show greater variability across different blending ratios. For 100% H2, the reaction R4 H+O2(+M)⇌ HO2(+M), which competes with R1, is the most important chain-inhibiting reaction in hydrogen combustion, showing the highest positive sensitivity. Additionally, the interconversion reactions between radicals such as H, O, OH, and HO2 also demonstrate a certain level of sensitivity.⇌ Figure 5.
Sensitivity analysis of IDTs for 100% H2, 50% H2/50% CH4, and 30% H2/70% CH4 under conditions of 1,100 K and 5.0 atm.
H2+OH⇌H+H2O (R4) CH3+HO2⇌CH3O+OH (R5) CH3+O2⇌CH2O+OH (R6) CH4+OH⇌CH3+H2O (R7) H+O2(+M)⇌HO2(+M) (R8) CH3+CH3(+M)⇌C2H6(+M) (R9) CH3+HO2⇌CH4+O2 (R10) C2H6+OH⇌C2H5+H2O (R11) As the methane proportion increases to 50%, in addition to the reactions mentioned above, methane combustion-related reactions begin to appear among the sensitive reactions. Particularly, reactions involving CH3, such as R5 CH3+HO2
CH3O+OH, R6 CH3+O2⇌ CH2+OH, R7 CH4+OH⇌ CH3+H2O, R9 CH3+CH3(+M)⇌ C2H6(+M), R3 CH4(+M)⇌ CH3+H(+M), and R10 CH3+HO2⇌ CH4+O2 become significant.⇌ For ignition-promoting reactions, in addition to the dominant role of R1, reaction R4 H2+OH
H+H2O is also an important chain propagation reaction that produces reactive H radicals, with a sensitivity coefficient higher than that under 100% H2 conditions. Reactions R5 and R6 also exhibit significant negative sensitivity. Among the positive sensitivity reactions, the chain-inhibiting reaction R7 converts active OH radicals into less reactive CH3, replacing R8 as the dominant inhibiting reaction for the 50% H2/50% CH4 mixture, with a slightly higher sensitivity coefficient than R8. Reactions R9, R3, and R10 are chain-terminating reactions in the methane combustion process, with R9 and R3 being pressure-dependent reactions that demonstrate higher sensitivity under elevated pressures. Reaction R9 consumes radicals through the combination of two CH3 species, thus inhibiting the ignition process, while reaction R3 suppresses ignition by consuming H and CH3. As the proportion of methane increases to 70%, the sensitivity of the carbon-involved reactions continues to enhance, as seen in R5, R6 CH3+O2⇌ CH2O+OH, R11 C2H6+OH⇌ C2H5+H2O. At this point, methane combustion gradually becomes the controlling mechanism of the system. In terms of inhibition reactions, chain-inhibiting reactions R7, R9, and R10, which are dominated by methane, begin to take precedence, while the chain-inhibiting reaction R8, which is primarily influenced by hydrogen, gradually diminishes.⇌ Figure 6 compares the peak concentrations of key radicals H, O, OH, and CH3 in 100% H2 and 50% H2/50% CH4. From the figure, it is clear that in the 100% H2 case, the concentrations of H, O, and OH radicals are relatively high, with no production of CH3. This indicates a strong ability to produce hydrogen atoms, reflecting the effectiveness of the chain-branching reaction R1. In contrast, in the 50% H2/50% CH4 mixture, the concentration of H atoms decreases sharply, while a certain amount of CH3 is formed. CH3 tends to consume active radicals, which together lead to a decrease in system activity, causing the IDTs to become longer.
Figure 6.
Peak concentrations of H, O, OH, and CH3 radicals at 1,100 K and 5.0 atm for 100% H2 and 50% H2/50% CH4.
Dilution gas effect
-
In oxy-fuel combustion and biogas combustion, there is a significant amount of CO2, which can have a noticeable impact on the IDTs of the fuel. The influence of CO2 and Ar on the ignition delay time of a 50% H2/50% CH4 mixture is shown in Fig. 7. From the figure, it can be observed that CO2 significantly increases the IDTs, indicating a negative effect on the ignition of the fuel mixture. Additionally, when the temperature drops below 1,538 K, the mixture does not ignite in the presence of CO2, whereas Ar allows the mixture to ignite over a broader temperature range and has minimal impact on the IDTs. This difference can be attributed to two main factors. First, CO2 has a higher specific heat capacity, causing it to absorb more heat after the reflected shockwave reaches the fuel, slowing down the temperature increase and thus affecting the ignition temperature. Second, CO2 may also participate in chemical reaction pathways at high temperatures, further influencing the combustion process.
The sensitivity analysis of IDTs for a 50% H2/50% CH4 mixture at 1,600 K in a high CO2 environment is shown in Fig. 8. Under high CO2 conditions, the main ignition-promoting reactions remain R1 and R4, which continue to play dominant roles. However, for inhibition reactions, the leading reactions shift from R7 and R8 (under argon conditions) to R7 and R12 in the presence of CO2. From reaction R12, it can be observed that CO2 directly participates in the reaction with H radicals, rather than just acting through collision effects. CO2 competes with O2 for H radicals, weakening the chain-branching effect of reaction R1, which leads to extended IDTs and reduces the reactivity of the fuel.
CO+OH⇌CO2+H (R12) -
This study conducted shock tube experiments and kinetic modeling to investigate the ignition characteristics of hydrogen combustion and hydrogen/methane co-combustion. The main conclusions are as follows:
(1) The IDTs of pure hydrogen remain low within the temperature range of 1,040−1,250 K, but it increases sharply at temperatures below 1,040 K. As the methane proportion increases, the IDTs curve shifts toward higher temperatures. When the fuel contains 70% methane, the ignition temperature significantly rises, and IDTs increase substantially. With increasing pressure, the IDTs curve shifts to lower temperatures, resulting in shorter IDTs. Comparing the IDTs results for different methane blending ratios indicates that hydrogen/methane co-combustion allows flexible adjustment of IDTs and the reactivity of the hydrogen fuel over a wide range.
(2) Different kinetic models perform well in predicting IDTs at atmospheric pressure for varying methane blending ratios. However, at 5.0 atm in relatively low-temperature regions, noticeable differences arise, particularly under pure hydrogen conditions. SJTU Mech, NUI Galway Mech, and USC Mech 2.0 models generally show better agreement with experimental data.
(3) Compared to pure hydrogen combustion, the addition of methane increases the concentration of radicals such as CH3 and decreases the concentration of radicals like H. This shift allows methane-dominated reactions to take control, particularly reactions such as R7 CH4+OH
CH3+H2O and R8 CH3+CH3(+M)⇌ C2H6(+M), while hydrogen-dominated reactions are weakened.⇌ (4) CO2 has a significant effect on ignition delay time. It directly participates in the reaction with H radicals and competes with O2 for H radicals, thereby weakening the promoting effect of reaction R1. This results in increased IDTs and reduced reactivity of the fuel mixture.
The research was supported by the fund of State Key Laboratory of Long-life High Temperature Materials (DEC8300CG202210287EE280292).
-
The authors confirm contribution to the paper as follows: conceptualization: Tian X; formal analysis: Tian X, Feng Z; investigation: Tian X, Liu S, Fang J, Zhang Q, Fang Q; writing - original draft preparation: Tian X; writing - review & editing: Liu S. All authors reviewed the results and approved the final version of the manuscript.
-
All data generated or analyzed during this study are included inthis published article.
-
The authors declare that they have no conflict of interest.
- Copyright: © 2024 by the author(s). Published by Maximum Academic Press, Fayetteville, GA. This article is an open access article distributed under Creative Commons Attribution License (CC BY 4.0), visit https://creativecommons.org/licenses/by/4.0/.
-
About this article
Cite this article
Tian X, Liu S, Fang J, Zhang Q, Feng Z, et al. 2024. Experimental and kinetic modeling investigation on ignition characteristics of hydrogen: effects of methane co-firing and dilution gas. Progress in Reaction Kinetics and Mechanism 49: e003 doi: 10.48130/prkm-0024-0002
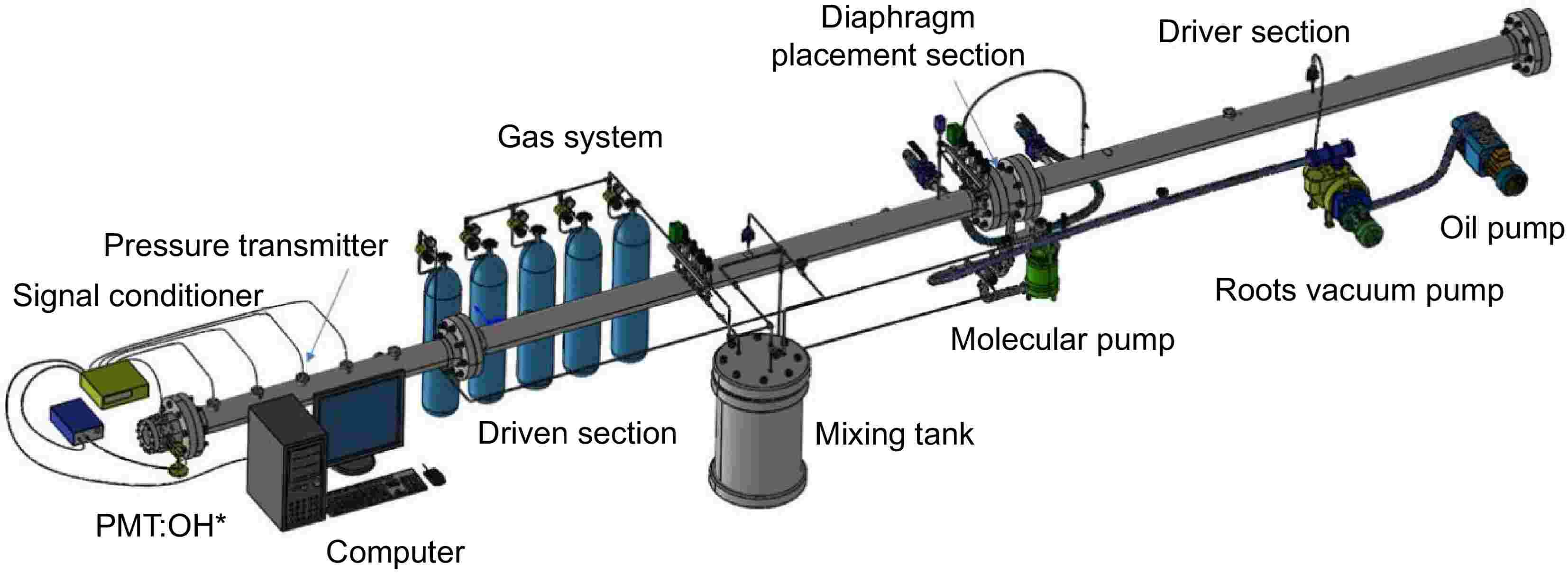