-
According to the survey by the China Edible Fungi Association, China is the largest country in the production and export of edible mushrooms[1,2]. Edible mushrooms are rich in minerals and are often considered an alternative source of meat, fish, and vegetables[3,4]. In particular, the demand for Pleurotus mushrooms is increasing year by year because of its high protein, dietary fiber, as well as essential and non-essential amino acids[5,6]. It has been known that each kilogram of mushroom can produce about 2.5−5 kg of the spent mushroom substrate (SMS)[7,8]. Currently, SMS is usually treated as agricultural waste and is landfilled, burned in the open on land, or composted with animal manure. These disposals may lead to environmental issues such as soil, air, and water pollution[9].
SMS is the lignocellulosic by-product of mushroom cultivation mainly referring to biomass waste which is not completely degraded as the degradation efficiency of edible fungi species only reached 40%−80%[10,11]. The term 'spent mushroom compost' (SMC) can be used interchangeably with SMS describing the agro-residues and fungal mycelium left after mushroom harvest[12]. SMS mainly consisted of residual fungal mycelium, various disintegrated lignocellulosic biomass (such as corn cob, sawdust, livestock litter and manure, cottonseed hull, wood chip, straw), amendments (such as lime, peat, gypsum), nutrients, as well as a high level of enzymes and organic matter[13−15]. A previous study has found that SMS has the characteristics of loose texture, small bulk density, rich particle structure, good air permeability, high nutrient, and water retention rate, which can effectively improve soil physical structure and soil microbial ecological environment[16].
After harvesting mushrooms, the SMS contains nutrients that are useful for crop, vegetable, fruit tree production and soil improvement[17]. Nowadays, there have been various studies using SMS as a biofertilizer to cultivate plants, including tomato, barley, pineapple, and so on. Lopes et al.[18] concluded that the use of SMS (Agaricus subrufescens) for seedling production had a higher total tomato production compared to previously reported production levels. Some experiments have also shown that cultivating pineapple in soil amended with SMS of oyster mushroom (Pleurotus ostreatus) increased its fruit number and optimized soil properties[19]. Field experiment results showed that the application of SMS at 50 and 100 t/ha significantly increased barley grain yield and soil nutrients, especially soil nitrogen and potassium content[20]. Vahid Afagh et al.[21] revealed that 10%–15% of SMS addition in the growing substrate significantly increased plant growth, flower yield, essential macronutrient uptake and soluble sugar content as well as essential oil percentages on German chamomile (Matricaria recursta L.) compared to the control treatment (no SMS). In addition, a previous report showed that SMS improved soil physical and chemical properties, and the speed of lawn establishment in turf production[22]. However, research on using SMS to cultivate forage has not yet been in-depth. Improving the nutrient content of grasslands in grassland management is one of the important measures to promote forage production. Therefore, exploring the impact mechanism of SMS on forage in degraded grassland soil can help the editable mushroom industry to achieve green development, transformation, and upgrading[23].
Alfalfa has been grown on approximately 30 million hectares for hay, silage processing and grazing globally[24]. With its long cultivation history and adaptability to a wide range of territories, alfalfa has been commonly used for animal feed[25]. It has also been utilized as a medicinal herb since it is a good source of vitamins (A, C, E, and K), protein, and minerals such as calcium, iron, potassium, and phosphorus[26]. Apart from these uses, alfalfa is an ideal natural resource and model plant for the remediation of contaminated soils, offering a variety of elite characteristics, including a highly productive biomass, drought tolerance, a fast-growing and prosperous root system, and availability in large amounts over several months of the year[27]. Based on the current cultivation status and application prospects of alfalfa mentioned above, it is quite important to study the application of SMS in alfalfa cultivation. Therefore, this research is based on the idea that SMS is expected to be used for alfalfa cultivation, filling the gap in this research field. Using SMS and soil mixed in proportion as a nutrient substrate. This research aims to explore the effect of SMS on the growth of alfalfa in degraded grassland soil, while improving the reuse efficiency of mushroom substrate.
-
The topsoil (0−20 cm) used in the experiment was taken from Duolun Restoration Ecology Research Station(42°20'N, 116°17'E), Inner Mongolian, China[28]. Soil properties were: pH 6.51, 84,066.46 mg/kg available nitrogen, 48.55 mg/kg dissolved organic carbon (DOC), and 232.79 mg/kg microbial biomass carbon (MBC). The type of experimental soil was classified as Haplic calisols according to the Food and Agriculture Organization of the United Nation (FAO) classification.
The experimental spent mushroom substrate (SMS) was the waste residue from 4−5 generations of Pleurotus ostreatus from Beijing Academy of Agriculture and Forestry Sciences, and its main components include 50% cottonseed hull, 40% corncob, a small amount of bran, and soybean meal (about 2%−5%). The spent oyster mushroom substrates properties were: pH 6.04, 90,650.61 mg/kg available nitrogen, 50,426.34 mg/kg DOC, and 50,626.21 mg/kg MBC. The spent mushroom substrate was thoroughly air-dried, crushed, and mixed before use.
Experimental design
-
The experiment included four substrate ratio treatments and one inorganic fertilizer with four replications. Therefore, these five treatments were 100% soil (CK), 90% soil and 10% SMS spent oyster mushroom substrates (10%SMS), 80% soil and 20% SMS spent oyster mushroom substrates (20%SMS), 70% soil and 30% SMS spent oyster mushroom substrates (30%SMS), and 100% soil with adding inorganic fertilizers (CF). Mix the crushed SMS and soil passing through a 2 mm sieve in proportion to each other and put them into disinfected pots one by one. Each plastic pot was filled with 0.4 kg dry weight of mixed substrate. According to previous studies, 200 mg/kg N and 30 mg/kg P (the actual fertilizer substances were (NH4)2SO4 and KH2PO4) were applied in the inorganic fertilizer treatment[29,30].
Use a 10% H2O2 (SCRC, Shanghai, China) solution to sterilize the surface of alfalfa (Medicago sativa L.) seeds with uniform size and full particles. Rinse them with sterile water several times and then sow them in plastic pots, with a few seeds per pot. Water them every two days. After the emergence of forage grass, eight plants per pot were established, cultivated in an artificial climate chamber with 16 h light, 8 h darkness, and a constant temperature of 25 °C. Under these conditions, alfalfa can have suitable growth conditions and good cultivation effects[31]. The experiment was conducted from April 2022 to December 2022 and harvested 40 d later.
Experimental method
-
This experiment measured the plants and soil of five treatments of alfalfa, including plant physiological indicators such as shoot biomass, root biomass, alfalfa nodule number, and total carbon (TC) and nitrogen (TN) of plants (including roots, stems, and leaves respectively), as well as soil microbial biomass carbon (MBC), microbial biomass nitrogen (MBN), dissolved organic carbon (DOC), and ammonium nitrogen (
-N), nitrate nitrogen (NH+4 -N), soil pH and available phosphorus (P).NO−3 We used scissors to cut aboveground alfalfa from the substrate surface, and then, the belowground was thoroughly washed with deionized water to remove soil particles. Shoots and roots were dried to constant weight at 65 °C and respectively weighed to measure the dry biomass of stems, leaves, and roots. Scan and calculate the number of alfalfa nodules using Epson Perfection V700 Photo (flat panel color image scanner) and WinRHIZO software. To measure TC and TN values of alfalfa components concluding roots, stems, and leaves by using Vario MACRO Cube Elemental.
We used the chloroform fumigation extraction method to determine MBC and MBN[32]. Five grams of two soil subsamples and incubate the non-fumigated group in a sealed cardboard box for 24 h (with water and NaOH solution inside). The fumigated group is vacuum filtered for 24 h (with water, CCl3, and NaOH solution inside) in a suction filter. After removal, add 20 ml of K2SO4 solution (0.5 M), shake and filter. Take 10 mL of the leaching solution and measure the data using an Analytik Jena Multi N/C3100 instrument to calculate the DOC, MBC, and MBN values.
Soil
-N andNH+4 -N were extracted from 10 g subsamples, added 50 mL KCl solution (1 M), shaken for 30 min, and then analyzed the filtrate with a continuous flow analyzer (AutoAnalyser 3, Analytical, Norderstedt, Germany)[33]. 1:2.5 soil/water suspension was measured substrates pH using Sartorius PB-10.NO−3 We used the molybdate-ascorbic acid method for the colorimetric measurement of available phosphorus[30]. Specifically, a 2.5 g air-dried substrate sample was added to 25 mL 0.5 mol/L NaHCO3 solution and about 1 g dephosphorized activated carbon, shaken at 20–25 °C for 30 min, and the suspension was filtered by double filter paper. Add 2.5 mL of molybdenum-antimony anti-mixed color reagent to 5 mL soil suspension, constant volume to 25 mL, shake and place at room temperature for 30 min. Available phosphorus concentration was determined using a multimode microplate reader (Varioskan LUX; Thermo Scientific, USA) at 880 nm wavelength.
Data analysis
-
Alfalfa growth data and soil indicator data were analyzed using one-way analysis of variance (ANOVA) by Duncan's multiple rang test and SPSS 20.0 software for processing; the significance level was p < 0.05. Before analysis, the Shapiro-Wilk test was used to evaluate the normality of the data. Levene test was used for analysis of variance to determine homogeneity of variance. Where necessary, the parameters were square root transformed to achieve normality and homogeneity. To explore the relationship between variables, the redundancy analysis (RDA) and Pearson correlation analysis were used for evaluation. All the figures were performed in R 4.2.1.
-
The shoot and root biomass of alfalfa cultured with 20% SMS, 30% SMS and CF treatments were significantly higher than those of CK treatment (Fig. 1, p < 0.05), but there was no significant difference between 10% SMS and CK treatments (p > 0.05). And there was no significant difference between the shoot and root biomass of 20% SMS and 30% SMS treatments compared with CF treatment (p < 0.05). CF treatment had the largest number of root nodules number, followed by 20% SMS, 30% SMS, and 10% SMS treatments, and CK treatment had the least number (Fig. 2). The number of nodules with 20% SMS and 30% SMS treatments was higher than CK treatment (Fig. 2, p < 0.05).
Figure 1.
(a) Shoot biomass, (b) root biomass and (c) picture of plant pot experiments of alfalfa under different treatments. CK: 100% soil; 10%: 10% SMS; 20%: 20% SMS; 30%: 30% SMS; CF: 100% soil with chemical fertilizers added. Different lowercase letters above the columns represent significant differences among these treatments according to Duncan tests.
Figure 2.
Number of alfalfa nodules under different treatments. CK: 100% soil; 10%: 10% SMS; 20%: 20% SMS; 30%: 30% SMS; CF: 100% soil with chemical fertilizers added. Different lowercase letters above the columns represent significant differences among these treatments according to Duncan tests.
TN and TC content of forage grass reflects its excellent quality. Our data showed that 20% SMS treatment had the highest TN and TC contents of alfalfa leaf (Table 1). The TN and TC contents of alfalfa root and stem were the highest with CF treatment, and there was no significant difference compared with 20% SMS and 30% SMS treatments (p > 0.05).
Table 1. Total carbon, nitrogen content, and carbon and nitrogen ratio (TC, TN, C/N) of alfalfa harvested in different treatments.
Treatment Root-TN (g) Root-TC (g) Root-C/N (%) Stem-TN (g) Stem-TC (g) Stem-C/N (%) Leaf-TN (g) Leaf-TC (g) Leaf-C/N (%) CK 0.53 ± 0.26b 8.05 ± 3.69b 15.44 ± 0.86b 0.27 ± 0.11b 7.27 ± 2.73c 27.17 ± 1.27a 0.73 ± 0.49a 12.51 ± 8.20b 23.32 ± 12.55ab 10% SMS 0.83 ± 0.28ab 14.13 ± 4.18ab 17.13 ± 1.09a 0.28 ± 0.09b 8.49 ± 2.96bc 29.99 ± 3.39a 0.98 ± 0.47a 23.49 ± 9.58ab 24.8 ± 3.63a 20% SMS 1.00 ± 0.30ab 17.71 ± 5.83a 17.56 ± 0.79a 0.52 ± 0.16a 14.58 ± 4.21ab 28.23 ± 2.71a 2.50 ± 2.16a 29.74 ± 7.79a 15.7 ± 6.43ab 30% SMS 1.04 ± 0.52ab 17.92 ± 8.80a 17.28 ± 0.57a 0.44 ± 0.19ab 13.16 ± 5.88abc 29.82 ± 2.14a 1.60 ± 1.09a 25.07 ± 17.19ab 11.76 ± 7.87b CF 1.16 ± 0.15a 19.77 ± 2.30a 17.13 ± 0.45a 0.58 ± 0.13a 15.53 ± 2.61a 27.78 ± 6.58a 1.93 ± 0.21a 29.06 ± 1.85ab 15.14 ± 1.13ab Different lowercase letters in the table represent significant differences among these treatments according to Duncan tests. Substrate physicochemical properties
-
The concentrations of DOC and available P significantly increased with the increase of SMS addition in the substrate (Fig. 3a & b, p < 0.05), and the concentrations were the lowest in the CK treatment. There was no significant difference between the 10%SMS and CF treatments (Fig. 3b, p > 0.05).
Figure 3.
Effects of different treatments on (a) soil dissolved organic carbon (DOC), (b) available P, (c) microbial biomass nitrogen (MBN), (d) microbial biomass carbon (MBC), (e) nitrate nitrogen (NO−3) and (f) ammonium nitrogen (NH+4). Means ± S.E. CK: 100% soil; 10%: 10% SMS; 20%: 20% SMS; 30%: 30% SMS; CF: 100% soil with chemical fertilizers added. Different lowercase letters above the columns represent significant differences among these treatments according to Duncan tests.
The MBN and MBC concentrations of 10% SMS, 20% SMS, and 30% SMS treatments were significantly higher than those of CF and CK treatments (Fig. 3c, p < 0.05). There was no difference between CF and CK treatments (p > 0.05).
concentrations of 10% SMS, 20% SMS, and 30% SMS treatments were significantly lower than that of CK and CF treatments (Fig. 3e, p < 0.05). NH4+ concentration was the lowest in 20% SMS treatment, while other treatments had no significant difference with CK treatment (Fig. 3d, p < 0.05). There was no significant difference in soil pH among all treatments (Table 2, p > 0.05).NO−3 Table 2. The pH of substrate with different treatments after harvesting.
Experimental treatment pH CK 7.12 ± 0.18a 10% SMS 7.08 ± 0.09a 20% SMS 7.06 ± 0.08a 30% SMS 7.06 ± 0.15a CF 7.10 ± 0.06a Different lowercase letters in the table represent significant differences among these treatments according to Duncan tests. Correlation analysis
-
RDA was used to assess how the physicochemical properties of the substrates influenced alfalfa growth indicators. It can be seen that there are significant differences in the substrate environment among different treatments, which have a significant impact on plant physiological indicators (Fig. 4). Plant physiological indicators respond strongly to the physicochemical characteristics of the substrate. The results show that the variance contribution rate of principal component 1 (PC1) and principal component 2 (PC2) respectively are 42.72% and 26.10%, and the cumulative variance contribution rate of PC1 and PC2 is 68.82%. Obtained through RDA diagram and calculation of Pearson correlation coefficient (PCC), available P had the strongest correlation with the PC1 and treatments distribution (r2 = 0.4035, p = 0.008), followed by root nodule (r2 = 0.2829, p = 0.047).
Figure 4.
The Redundancy Analysis (RDA) shows the effects of five experimental treatments on physical and chemical properties in substrate including pH, dissolved organic carbon (DOC), microbial biomass nitrogen (MBN), microbial biomass carbon (MBC), NH+4 and NO−3, available P (P) and plant indicators including shoot biomass, root biomass, plant total nitrogen (TN), total carbon (TC), and carbon nitrogen ratio (CN). The red arrow in the figure represents plant growth indicators, and the blue arrow in the figure represents physical and chemical properties in substrate.
The Pearson correlation analysis showed that the shoot biomass value of alfalfa was significantly correlated with the available P in the substrate (Fig. 5, p < 0.05). MBC and MBN were strongly correlated with DOC and available P (p < 0.05). Meanwhile, there is a strong correlation between DOC and P (p < 0.001).
Figure 5.
Correlation heatmaps of alfalfa growth indicators (shoot biomass and root biomass) and of nutrient concentration indicators in substrates (NO−3, NH+4, MBC, MBN, DOC, pH and P). NO−3: nitrate nitrogen; NH+4: ammonium nitrogen; MBC: microbial biomass carbon; MBN: microbial biomass nitrogen; DOC: dissolved organic carbon; pH: acidity and alkalinity of substrates; P: available P. The color intensity in each panel indicates the relative correlation between read numbers of two groups. Blue represents positive correlation, and red represents negative correlation. Statistically significant correlations are indicated with *p < 0.05, **p < 0.01, and ***p < 0.001.
-
SMS has been increasingly used as soil amendment either freshly or after composting processes in recent years[34]. The current study found that adding appropriate amounts of SMS to degraded grassland soil can promote the growth and quality of alfalfa. The shoot biomass of alfalfa in 20% SMS and 30% SMS treatment significantly increased respectively by 1.67 and 1.77 times compared to CK treatment (Fig. 1). 20% SMS treatment had the highest TN contents of alfalfa stem and TC contents of alfalfa leaf (Table 1). This result supports previous studies, which illustrated that sometimes organic manure amendment promoted plant growth more efficiently byproviding a higher nutrient value compared with inorganic fertilizer[35].
We found that our SMS materials contain far more nutrients needed by plants than soil, which means that adding SMS to degraded grasslands with poor soil is beneficial for plant growth. As an important factor affecting soil quality, soil nutrients typically can maintain soil quality and promote plant productivity[36]. During the growth process of Pleurotus ostreatus, due to the need for autotrophic nutrients, enzymes are secreted to mineralize the nutrients in the substrate, such as laccases, cellulases, hemicellulases, and xylanases[37]. Some of these nutrients are absorbed by the mushrooms themselves, while others are left in the substrate, such as carbohydrates, protein, and fat[38]. So, SMS contains different levels of carbon and nitrogen higher than soil, which can improve the storage capacity of soil nutrients when applied as substitutes for fertilizers[39]. The results of our research concluded that the soil application of SMS improved soil fertility since the DOC and available P were increased significantly (Fig. 3). When applied as an alternative fertilizer, the nitrogen in SMS can be slowly released into the soil, which is beneficial for plants to assimilate nitrogen[40]. And SMS is rich in phosphorus, which can be used as a phosphorus additive in soil to increase soil organic matter and nutrient content[41,42]. Besides, the rich organic matter in SMS improves soil structure, soil aeration, and water retention, and even increases soil microbial activity[43]. The increased organic C and available N in soils amended with organic manure would provide more organic resources that benefit the microbial activity in soils[44]. Based on the previous research, four months after the addition of Agaricus bisporus SMS to the soil, organic N and available P content of the soil increased, while the impact on pH is not significant[7], which is consistent with our experimental results. The effectiveness of SMS as an organic soil amendment has also been positively evaluated by Courtney & Mullen[20]. The effect of applying SMS was equivalent to fertilization, where the C, N and P contents in the soil respectively increased by 40%, 28%, and 230%, meanwhile, the calcium, potassium, and magnesium content increased by three times.
In addition, SMS increased the beneficial microbial biomass in the substrate, which may be one of the reasons why SMS promotes plant growth. A previous study has found that Rhizobia is beneficial to leguminous plants and can effectively fix nitrogen in the air for plant use[45]. Our data showed that the number of nodules increased with the increase of SMS addition, which can be further inferred to increase the effect of nitrogen fixation (Fig. 2). The mutually beneficial relationship between symbionts and host plants is established of based on an 'investment-return' strategy balance, which means that alfalfa uses rhizobia to obtain nutrients while correspondingly spending energy to maintain microbial life[46]. Although the number of nodules in CF treatment was significantly higher than in other treatments (Fig. 2), there was no significant increase in biomass compared with 20% SMS and 30% SMS. It is possible because higher levels of nitrogen or phosphorus would break potential nutrient limitations, allowing host plants to easily obtain essential nutrients for growth through their roots, and plants would invest fewer resources in rhizobia at this point[47]. The MBN and MBC concentrations of adding SMS treatments were significantly higher than no SMS treatments (CK and CF), which indicated that SMS addition promotes microbial biomass, while inorganic fertilizer addition does not have such effects (Fig. 3c & d). Research has shown that the different microbial populations of bacteria and fungi carried by SMS also can degrade organic foreign compounds in soil[48], which may promote plant absorption of chemical fertilizers. Additional research instructed that microorganisms play an important role in soil fertility and nutrient cycling[49]. Therefore, SMS can promote the formation of root nodules, and increase the N content and the biomass of alfalfa.
Correlation analysis indicated that the soil available P concentration and the number of alfalfa nodules significantly affect the growth of alfalfa (Fig. 4). The shoot biomass of alfalfa was significantly correlated with the soil available P concentration, and MBC and MBN have a strong correlation with DOC content, and DOC is also related to P content (Fig. 5). Alfalfa has high requirements for phosphorus, sulfur, potassium, and some micronutrients, such as boron, and if one nutrient is deficient in soil, crop growth will be poor even if other nutrients are abundant[50]. Therefore, it is inferred that the rich organic carbon and high microbial activity in the SMS improve nutrient cycling within the substrate, and the high P content in the substrate promotes an increase in alfalfa biomass.
-
The experimental results showed that the shoot and root biomass, DOC, available P, MBC, and MBN concentrations of alfalfa enhanced with the increase of SMS addition in degraded grassland soil. Among them, 20% SMS and 30% SMS treatments had no significant difference in alfalfa biomass compared with CF treatment. 20% SMS treatment had the second highest number of alfalfa nodules only after the CF treatment. Total carbon content in leaves and total nitrogen content in stems of 20% SMS treatment were significantly higher than other treatments. The available P was significantly correlated with shoot biomass, MBC, MBN, and DOC. In conclusion, the effects of 20% SMS treatment on alfalfa growth and soil improvement were superior to other treatments. Therefore, adding 20% SMS to the degraded grassland soil to cultivate alfalfa has a good economic and application prospect, which can improve the yield and quality of alfalfa, promote the recycling of waste and reduce the waste of resources. This study provides a theoretical basis and support for the large-scale application and popularization of this technology in the field.
-
Thanks to the College of Grassland Science and Technology at China Agricultural University for their guidance and assistance in this experiment. Also thanks to the Beijing Academy of Agriculture and Forest Sciences and Duolun Restoration Ecology Research Station, Institute of Botany, and Chinese Academy of Sciences for their material support in this experiment. Thanks to Hengkang Xu, Lu Lian and Bin Wei for their support in this project.
-
The authors declare that they have no conflicts of interest. Yingjun Zhang is the Editorial Board member of Grass Research who was blinded from reviewing or making decisions on the manuscript. The article was subject to the journal's standard procedures, with peer review handled independently of this Editorial Board member and his research groups.
- Copyright: © 2023 by the author(s). Published by Maximum Academic Press, Fayetteville, GA. This article is an open access article distributed under Creative Commons Attribution License (CC BY 4.0), visit https://creativecommons.org/licenses/by/4.0/.
-
About this article
Cite this article
Shi Y, Cui X, Zhang Y, Liu M. 2023. The addition of spent oyster mushroom substrates has positive effects on alfalfa growth and soil available nutrients. Grass Research 3:19 doi: 10.48130/GR-2023-0019
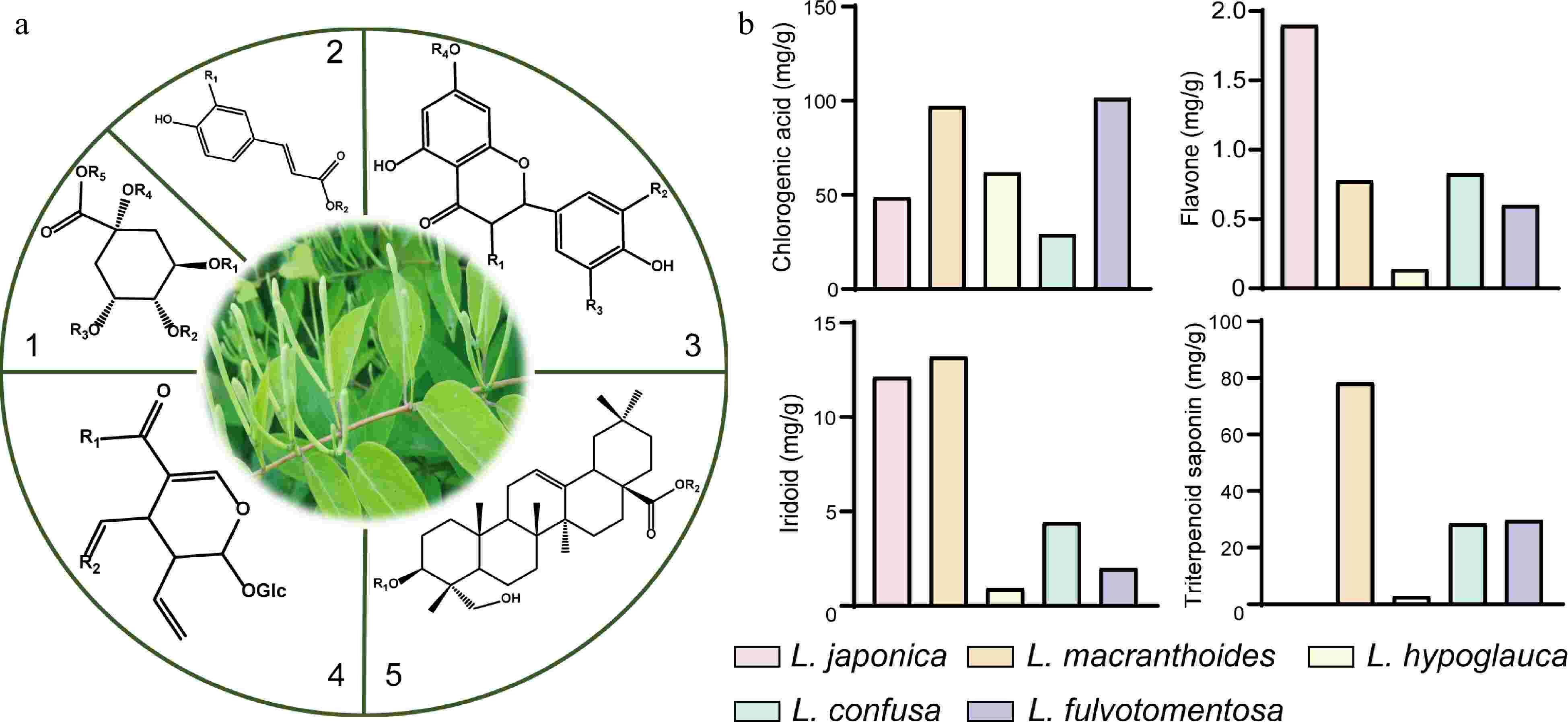