-
Pome fruit, namely the domesticated apple (Malus domestica Borkh.) and European pear (Pyrus communis L.), constitute one of the major classes of fruit crops in terms of world fruit production and represent the majority of cultivated temperate fruit species in the Rosaceae family[1]. During the yearly endodormancy phase in the winter period, these trees require an annual cold period below 7 °C to break chilling requirements, which is necessary for successful growth and flowering in the following season[2]. However, upon this growth resumption, frost periods in temperate areas are increasingly common and can damage floral tissues[3]. Pome fruit trees initiate their reproductive cycle during early spring, and consequently developing flower buds are often exposed to occasional freezing events during spring. During these spring frost events, ice nucleation can result in cell damage or lysis[4]. With climate change significantly increasing the severity and periods of extreme late-spring frosts in many pome fruit-producing regions, developing floral organs are increasingly at risk after breakage of endodormancy, with high occurrences of disrupted flower development and even floral abortion. As a result, frost damage can result in reduced fruit set, making frost tolerance of reproductive tissues an important trait in pome fruit production[5]. Frost tolerance, in general, refers to the capacity of tissues to survive during chilling at temperatures ranging from 0 to 18 °C as well as under freezing conditions, i.e., below 0 °C[6,7].
Late spring is the critical period during which flowers are maturing and constitutes a focal point for the success of commercial fruit production. Cultivars sensitive to late-spring frost may not form functional reproductive cells (both male and female gametes) as the sporogenesis or gametogenesis process may be inhibited, or floral organs may be destroyed or aborted upon exposure to frost[8,9]. This can eventually lead to substantial reductions in fruit yield, making late-spring frost one of the major causes of global temperate pome fruit losses[8,9]. For example, in 2017, apple and pear production in the European Union were 9.3 million tonnes and 2.1 million tonnes, respectively, decreasing 21% and 1% compared to 2016 due to severe late-spring frost[10]. This yield loss was mainly attributed to the exposure of floral tissues to sub-zero temperatures during the most sensitive stages of reproductive development between late autumn and spring[9]. Early frost, occurring before the endodormant bud stage in autumn can destroy the whole bud tissue, whereas late frost events after bud burst in spring are particularly damaging to specific floral tissue types or maturing flowers[8,11]. Apple and pear flowers at the full pink and white stages, respectively, exposed to −2 and −4 °C for 30 min on average suffer from 10% and 90% flower kill[9,11]. To quantify frost damage, fruit setting can be measured as an indicator, avoiding biotic and abiotic factors that may impact yield later in the season.
In this context, frost tolerance of developing flowers and particularly all reproductive organs (stamen, stigma, and ovules) during late spring are an important factor determining the environmental resilience of apple and pear trees and is therefore becoming a key breeding trait[12]. To overcome economic losses caused by late-spring frost, freezing-tolerant pome fruit germplasm should be developed, although to date only limited evidence for this has been reported[12−14]. A few apple breeding programs have already focused on this trait and have led to the development of some successful germplasm lines. For instance, a set of diploid and triploid Russian apple cultivars and American apple rootstock cultivars such as 'Geneva® 935' demonstrated particularly high frost tolerance[13,14]. Assessing the actual level and natural genetic variability of frost tolerance of reproductive organs is a key aspect of efficient breeding for enhanced cold resilience in pome fruit crops, with its targeted improvement requiring an accurate determination of key tolerance components for reliable selection and cultivar development.
The current scientific methodology to assess frost tolerance in pome fruits is fractional and unstandardized. Particularly, current evaluation methods for frost tolerance mainly encompass simple visual field studies or occasionally include more advanced methods like electrolyte leakage or differential thermal analysis (DTA). These methods are limited as they are unreliable or involve laborious measuring techniques[15,16]. In situ field evaluations and molecular studies like electrolyte leakage rely on the natural occurrence of frost events in the orchard and are therefore hindered by unpredictable weather conditions that are linked to the geographical area, often restricted to the northernmost global regions[6,9,10]. Attempts to visually evaluate the freezing tolerance of reproductive tissues in pome fruit species focus on evaluating flower buds and organ damage at different stages of floral development[9,17], but often fail due to evaluation biases and environmentally dependent variation within observation plots. In contrast, DTA determines the frost tolerance by evaluating multiple tissue samples processed in multi-cell trays through which heat flow is measured at discrete temperature scales (often decreasing below freezing) against a reference, resulting in a thermogram. From these thermograms, inferences on frost tolerance are made by assessing the response of the tissue to discrete temperature levels[15,16]. Following DTA, processed samples are immersed in a buffer and measured for electrolyte leakage to indicate cellular damage post-freeze. DTA is a standard method for assessing tissue frost damage, although it is highly laborious as it requires multiple unique samples to be run at discrete, stepwise temperatures (e.g., −10, −20, −40, and −80 °C), with a single run typically taking up to 22 h. In addition, as DTA generates only discrete temperature data for each sample, it has relatively low discriminatory power[18]. Despite being already used for monitoring frost tolerance, these methods rely on various time-dependent and laborious protocols, which make precise, high-throughput evaluations of the freezing temperature of floral samples or other plant tissues practically challenging and unreliable[15,16,18] .
An alternative and more accurate approach to determining frost tolerance of plant tissues is differential scanning calorimetry (DSC). This method can simulate natural in situ freezing conditions and not only determines the freezing point, the condition at which tissues freeze but also the supercooling window, i.e., the conditions in which liquids do not freeze at normal physiological points. DSC reveals the exact occurrence of the phase transitions of a tissue by monitoring both exo- and endothermic heat flows while cooling or heating, relative to a control reference[19]. DSC has already been applied to stone fruits to determine the supercooling and freezing points of flowers, buds, and stems of apricot (Prunus armeniaca) by cooling the different tissues from 5 to −7 °C at a rate of 2 °C/h[20]. This study demonstrated that DSC enables accurate and consistent measurement of the freezing temperature of specific floral organs of three Prunus cultivars, which allowed ranking them according to their respective frost tolerance. DSC has also been applied to a frost tolerance study in whole plants of Arabidopsis thaliana[21]. This revealed that DSC is a useful tool in exploring Arabidopsis leaf frost tolerance by measuring heat flow and freezable water (fH2O, i.e., supercooling capacity) in the leaves while cooling and reheating them across a 25 to −18 °C temperature window, at a rate of ± 10 °C/min[21]. The DSC method therefore provides an easy and straightforward tool to refine and accurately determine the frost tolerance of plant floral organs, and perhaps other tissues such as terminal shoots[20]. However, this technique is yet to be tested for determining frost tolerance of reproductive tissues in pome fruits.
Here, we explore the DSC analysis approach to determine the frost tolerance of different floral tissues, i.e., stamens, stigmas, and ovules of 'Jonagold' apple and 'Conference' pear trees by measuring both their internal freezing and melting points and supercooling capacity under a controlled cooling-heating treatment.
-
Two-year-old branch pieces with just open king flowers were taken from the stems of adult 'Jonagold' apple and 'Conference' pear trees located in experimental orchards in Gingelom and Herk-de-Stad, Belgium, in April 2019. Immediately after sampling, the wood with flowers was placed in distilled water and transported to the laboratory for tissue sample preparation and DSC measurements. First, the open king flower, being the most mature flower within a flower cluster, was detached from the branch part using a sterile scalpel. Specific floral organs directly involved in the generation and transmission of gametes, i.e., ovules, stamens, and stigmas, were excised with a sterile scalpel, weighed, and inserted into a hermetically sealed aluminum calorimetric pan (TA Instruments, LLC, New Castle, DE, USA). The pan was then placed into the DSC Q2000 device (TA Instruments, LLC, New Castle, DE, USA) together with an empty reference pan. Samples were held at 20 °C for 1 min before cooling to −40 °C at 10 °C/min, kept at that temperature for 1 min and finally heated back to 20 °C at 10 °C/min. The entire cooling/heating cycle was repeated once on the same sample to check for reproducibility. For each tissue type, DSC measurements were performed on nine to 12 biological replicates. Scanning rates of 10 °C/min were used to enable high-throughput DSC assaying of the floral tissues. Tissues were observed with light microscopy at 20 °C, −40 °C, and 20 °C, under conditions similar to the first DSC run using an Olympus BH2 optical microscope, equipped with a ProgRes® CF camera (Jenoptik Optical Systems GmBH, Germany) and a Linkam optical DSC600 Hotstage for temperature control at a rate of 10 °C/min to confirm that the tissue integrity has not been compromised. DSC records the heat generated during exothermic ice formation, which is maximal when the crystallization rate is highest (Fig. 1 & Supplementary Fig. S1). The crystallization onset temperature of plant tissues in a DSC setting (i.e., in the range from −10 to −15 °C) is lower than in an orchard setting (around −2 °C)[9] due to the high cooling rate. To keep sampled flower tissue fresh in the lab and to establish a high-throughput validation of this method, 10 °C/min was chosen as the cooling rate. In each cooling/heating run, three characteristic transition temperatures were extracted: the onset of ice crystallization, the maximum crystallization rate (the peak value), and the melting point, respectively. These temperatures are referred to as the breaking point ('Breaking'), the crystallization point ('Cryst'), and the melting point ('Melt'), respectively. A total of six data points per sample were recorded during measurements (one set of three labelled as point Pt1 and the other as Pt2). Two sets of measurements were individually recorded by two consecutive cycles.
Figure 1.
Differential scanning calorimetry (DSC) heat flow plot of a pear ovule generated by the TA Instruments Q2000 DSC machine, representing two cycles of cooling and heating, each with three main characteristic data points (breaking points, crystallization point, and melting point). Numbers 1 and 2 at each data point represent the first and second cycle (cycles A and B), respectively.
Statistical analysis
-
DSC data of all tissue samples acquired during the two freezing and melting cycles at the three measuring points (breaking, crystallization, and melting points) were subjected to a multivariate principal component analysis (PCA) using the R statistical interface to determine the principal component loadings that explain data variance for each measuring point. The values for the selected measuring point of the first cycle, i.e., breaking, crystallization, and melting point 1, were then subjected to a Shapiro test (shapiro.test) and a visual evaluation of the distribution plot for testing of normality (Supplementary Fig. S2). Normally distributed temperature data of the first freezing and thawing cycle of 'Jonagold' and 'Conference' sample groups was statistically analyzed using the standard analysis of variance methodology (ANOVA; aov) followed by a post-hoc Tukey test (TukeyHSD) for pairwise statistical comparison. The equality of the measuring points across the two cycles was compared using the F test that compared variance among different measuring points.
-
DSC measurements at six different data points enabled selection of data points of the flower tissues for both 'Jonagold' apple and 'Conference' pear for further analysis of frost tolerance (Fig. 2 & Supplementary Table S1). PCA based on all six data points showed that samples from the two pome fruit species (pear and apple) partially overlapped for each of the three tissues analyzed, suggesting that some differences in freezing tolerances may exist between ovules, stigmas, and stamens, as well as between the fruit species (Fig. 2a). In particular, ovule samples formed groups that clustered completely separately from the groups of stamen samples. Regarding DSC temperature peaks, points marking the onset of ice crystallization during the first and the second temperature cycling, i.e., Breaking_Pt1 and Breaking_Pt2, respectively, and data points corresponding to the point of ice crystal formation, i.e., Cryst_Pt1 and Cryst_Pt2, showed similarly high loading scores along the first principal component (Fig. 2b & Supplementary Table S2), which explained 64.2% of the total variance (Fig. 2a). In contrast, the data points corresponding to the ice melting point in the samples, i.e., Melt_Pt1 and Melt_Pt2, resulted in high scores along the second principal component (Fig. 2b & Supplementary Table S2), which explained 29.1% of total variance (Fig. 2a). Crystallization onset (Breaking_Pt1) was chosen for the comparative analysis of freezing tolerance, as a mean temperature was not significantly different between the four analyzed freezing data points (Breaking_1, Breaking_2, Cryst_1, and Cryst_2) (p > 0.31) and all four data point show significant correlation among themselves and between the cycles (Supplementary Fig. S3). Melt_Pt1 was chosen for the analysis of the sample thawing process.
Figure 2.
(a) Principal component analysis (PCA) plot showing variance distribution of the six different differential scanning calorimetry (DSC) data points of all apple and pear floral organ samples according to principal component 1 and 2 (PC1 and PC2) with sample grouping according to tissue (ovule, stamen, or stigma) and species type (cultivars 'Conference' pear and 'Jonagold' apple) as indicated by the ellipsoid figures. (b) PCA loading plot showing the relative contribution of the different DSC data points to the first two principal components of the PCA analysis of all samples. PC1 is determined by Cryst_Pt1, Cryst_Pt2, Breaking_Pt1, and Breaking_Pt2 data points, whereas PC2 is determined by Melt_Pt1 and Melt_Pt2.
Using the temperature at the onset of crystallization, i.e., Breaking_Pt1, for the statistical comparison of the onset of crystallization, significant effects were found for both species type (pear vs apple) and floral tissue, together with a significant interaction between both parameters (p values of 0.042, 8.76e-05, and 7.26e-06, respectively). These effects were reflected in the pairwise comparison of specific tissues between the two species, as well as when comparing tissues within a specific species (Fig. 3a). The 'Conference' pear genotype had a lower freezing onset temperature for stamens (−13.1 °C) as compared with the 'Jonagold' apple (−11.1 °C), but a higher temperature for ovules (−9.6 °C vs −11.3 °C). In contrast, the freezing onset temperatures of stigmas did not differ between pear (−11.6 °C) and apple flowers (−11.0 °C) (Fig. 3a & Supplementary Table S1). When comparing the freezing tolerance of the different floral organs within each pome fruit species, significant differences were observed between all three organs in pear, with stamens showing a lower point compared with stigmas and ovules. Such differences, however, were not observed across the different flower organ types in apple, with stamens, stigmas, and ovules all showing a crystallization onset temperature averaging around 11 °C. Analysis of ice melting, i.e., Melt_Pt1, showed that different tissue types, tree species, and the interactions between the two parameters affect the melting temperatures (p values < 2.02e-08, respectively). Apple stamens thawed at the highest temperature (4.8 °C), followed by the stigma and ovules (2.9 and 1.1 °C, respectively). Pear stamen and stigma thawing temperatures were lower compared to 'Jonagold', i.e. 2.5 and 2.2 °C, respectively, whereas ovules thawed at the lowest temperature among different tissues (1.5 °C), but this did not differ from the thawing temperature of 'Jonagold' ovules. Visual assaying of 'Jonagold' reproductive organs during the DSC freeze-thaw cycle revealed a distinct sample tissue discoloration from lighter to darker because of ice crystal formation from 20 to −40 °C, which reversed back to lighter shades during the thawing step towards 20 °C, and did not result in any obvious tissue degradation (Fig. 3b).
Figure 3.
(a) Pairwise comparison of the freezing (Breaking_Pt1) and thawing (Melt_Pt1) temperatures of different floral organs, as measured via differential scanning calorimetry (DSC) analysis during the first cooling-heating cycle of the pear cultivar 'Conference' and the apple cultivar 'Jonagold' (n ≥ 9; ± SE; Tukey p ≤ 0.05). Significant differences in freezing temperature among tissue types for each species are indicated by different letters. (b) Light microscopy pictures of ovules (top), anthers (middle), and stigmas (bottom) of the apple cultivar 'Jonagold' taken while temperature cycling in the microscopy chamber from 20 to −40 °C (first and second picture of each sample) and after the thawing (last picture of each sample).
-
This study showed that DSC can be used to compare freezing points of different types of floral organs in apple and pear without interference from environmental effects, therefore reflecting physiological differences in freezing temperatures, although not necessarily reflecting the exact freezing temperatures from the field. This is the first time that differences in freezing points have been studied at the level of individual floral organs and reproductive tissues of pome fruits. The observed variability in freezing temperature among floral organs of 'Jonagold' apple (from −11.0 to −11.3 °C) and 'Conference' pear (from −9.6 to −13.1 °C) corroborates previous reports where differences in freezing tolerance of whole flower buds were observed between apple and pear. More specifically, floral buds from the apple cultivar 'Red Delicious' were reported to freeze from −6.8 to −11.8 °C[11] and from −5 to −12 °C in Pyrus pyrifolia[22]. Similarly, the freezing and breaking temperatures of stamens were consistently lower compared to the corresponding temperatures of stigmas, which is in line with the results from apricots[20] and sweet cherry[23,24]. The strong variability in frost tolerance observed in these studies likely reflects development-dependent differences and may also be attributed to technical variation, such as biases in the DTA methodology and visual assessment of frost damage. DSC enables more accurate measurements, but the measured freezing temperatures can differ from those observed in fruit growing practice[9,17,25]. This is because that during the DSC analysis samples are supercooled at an accelerated rate compared to the field, implying that the tissue temperature is additionally dropped before the formation of ice crystals. Plant supercooling is a physiological response in which plants avoid water crystallization on a cellular level by increasing solute concentrations within cells[26]. Similar supercooling conditions can occur during natural frost events in the field, particularly during late spring frost in apple and pear orchards[27,28]. For example, a large freezing event occurred in April 2008 in Yakima, Washington, USA, the largest apple-producing region in the USA[29]. During this period, several short-term field temperature events below 0 °C occurred within 18 d, where the average daily temperature change was ± 13.7 °C (PRISM Climate Group, April 2008). Although the artificial freezing program during DSC analysis exceeds natural cooling rates and frost levels occurring in the field, the use of this method allows validation of frost tolerance of pome fruit tissues and organs via identification of the exotherm and endotherm peak values, representing the exact physiological freezing and thawing temperature, respectively. Calibration of the obtained temperatures and temperature cycling rates to further align and normalize them with those in the field is required in the next parallel experiments under both standardized conditions (e.g. in a phytotron) and field conditions.
Multiple temperature cycling runs can be performed using DSC, as was done here, to assess hardiness over repetitive but separate frost events in the field. Though we did not explore this metric, it is evident that the freezing point mostly increased in the second run (Supplementary Table S1). This difference could reflect increased susceptibility to frost and freezing damage upon the first freezing and thawing cycle. It might occur due to tissue- and flower-specific damage and cell lysis as a consequence of intra- and inter-cellular ice crystal formation that can disrupt cell integrity. Resilience to this freezing-thawing process might be particularly important during warmer winters when plants lose their dormancy and become physiologically active. In this regard, thawing temperature should be considered as well. We identified the stamens of 'Jonagold' apples to have the highest thawing temperature. Identification of plants with relatively high thawing temperatures of the tissues exposed to frost would be instrumental in the identification of frost-hardy plants. Though, not only flowers but also other tissues could be considered, as bark can suffer from sun scald particularly during warm winter days[30]. In this regard, correlations between DSC-observed values upon reoccurring freezing and thawing, and field values must still be explored in different fruit species and organs, particularly with solute concentrations and environmental events in mind (e.g., flower position).
This study identified several improvements of DSC over currently available techniques for measuring the freezing temperature point in pome floral tissues, such as DTA, electrolyte leakage, and visual evaluation of frost damage. DSC enables high-throughput assaying of tissue freezing temperatures, with a single sample run taking less than 30 min. During that time precise freezing and thawing temperature points, as obtained from continuous exothermic and endothermic signals, respectively, are determined only in a single run. However, like DTA and electrolyte leakage, DSC also comes with some drawbacks. These include limitations in sample volume and weight, i.e., largely depending on the DSC device and chamber size, difficulties in storing samples post-freeze, and restrictions linked to single sample running. However, some of these drawbacks can be overcome by using the described methods in newer devices with multiple chambers such as the TA Instruments Multi-Sample X3 DSC, allowing high-throughput and simultaneous analysis of multiple samples in parallel[31]. Although we attempted to visualize the frost damage by performing real-time microscopy imaging, one possibility to enhance the quality of DSC analysis would be to combine it with electrolyte leakage assays. Electrolyte leakage allows quantification of the degree of frost damage on a cellular level by measuring the number of free ions resulting from cell rupture (e.g., K+, Ca2+), complementing the determination of freezing points by DSC. Another way to improve the accuracy of the DSC methodology is to integrate measurements of osmotic potential as this is directly affected by freezing damage. If osmotic potential (solute potential) can be quantified, DSC appears a promising high-throughput replacement for DTA and electrolyte leakage studies.
This study shows that DSC offers an easy method to characterize the freezing temperatures of reproductive tissues in new and existing pome fruit germplasm, with potential opportunities for genetic studies and breeding. Currently, frost tolerance data for existing and new cultivars and their floral tissues are generally not known, and if they are, they exhibit large variability[11,13,32]. With the increased occurrence of detrimental frost events during late spring due to global climate change, insights into this physiological parameter are becoming increasingly important and frost tolerance is recognized as a key trait for sustainable pome fruit breeding. To overcome this knowledge gap, DSC appears to be a valuable tool, as it can be immediately applied to different tissue and organ types, such as vegetative and reproductive buds as well as floral organs, at different developmental stages for a broad range of individual genotypes or cultivars. Furthermore, DSC can be coupled with advanced biochemical and molecular approaches to further characterize the biological mechanisms underlying freezing point determination and to search for new methods to enhance the freezing tolerance of floral buds or other tissue types. This includes the quantification of specific plant proteins, such as candidate anti-freeze glycoproteins (AFGPs) and thermal hysteresis proteins (THPs)[33], as well as metabolites such as xylitol[34,35]. An immediate candidate metabolite for profiling is proline, a potent osmoprotectant known to play key roles in conferring tolerance to freezing[36,37] as well as to drought[37,38]. Additionally, DSC may facilitate studying the effect of grafting frost-susceptible scions on cold-hardy rootstock varieties. This is critical for fruit breeders since rootstock-scion interactions are presumed to form a versatile and straightforward approach to enhance the overall frost tolerance in orchards[14,39,40]. To explore the usefulness and limitations of DSC as an indicator of frost tolerance, robust DSC models correlated with in situ field conditions and phytotron freezing studies should be conducted and compared to field freezing data under similar conditions.
-
DSC was explored as a standardized and high-throughput method for accurately evaluating the frost tolerance of apple and pear floral organs in super-cooling conditions. Our study showed that DSC enables efficient determination of freezing points by quantifying the 'crystallization' and 'breaking' points, thereby revealing differences in frost tolerance between species (apple vs pear) and some cases amongst floral organs, i.e., stamens, stigmas, and ovules. In particular, floral tissues of the pear cultivar 'Conference' showed significant differences in freezing temperature, whereas this was not observed for the apple cultivar 'Jonagold'. Similarly, thawing temperature differences were identified among different tissues and species, which could reflect resilience to multiple freezing-thawing cycles. The obtained findings are largely consistent with previous data obtained using other techniques, such as DTA, but potentially offer a more high-throughput, time-efficient alternative to measure freezing points with greater accuracy due to continuous data collection during thermal cycling. If sufficient data is obtained from DSC and correlated with phytotron and field frost tolerance values for individual genotypes, this method may aid in breeding new varieties less susceptible to late spring frost damage.
The authors acknowledge Tine Verhoeven, Kristina Ilnikar, and Ide Buwalda for their help in sampling, sample preparation, and data acquisition. Anže Švara was a Research Foundation Flanders (FWO) Aspirant grant holder during the execution of this experimental work (Grant No. 1161518N).
-
The authors confirm contribution to the paper as follows: study conception: De Storme N, Keulemans W, De Maeyer L, Goderis B, Deckers T; experiments conducting: Švara A, Deckers T, De Maeyer L, Colaers M; data analysis and draft manuscript writing: Švara A, Upchurch D. All authors reviewed the results and approved the final version of the manuscript.
-
The datasets generated during and/or analyzed during the current study are available from the corresponding author on reasonable request.
-
The authors declare that they have no conflict of interest.
-
# Authors contributed equally: Anže Švara, Davis Upchurch
- Supplementary Table S1 Summary of measuring points by cultivar and floral tissue.
- Supplementary Table S2 Principal component (PC) loadings for each measuring point.
- Supplementary Fig. S1 Examples of differential scanning calorimetry (DSC) heat flow plot for apple and pear ovule generated by the TA Instruments Q2000 DSC (TA Instruments, New Castle, Delaware, USA.) representing two cycles of cooling and heating to illustrate diversity of plots obtained by this method.
- Supplementary Fig. S2 Density and qq plots for the recorded temperature data (i.e. breaking, crystallization, and melting points) of the first freezing-thawing cycle. "p" shows the Shapiro-Wilk p-value for each measured data point.
- Supplementary Fig. S3 Correlation plots for the recorded temperature data points (breaking, crystallization, and melting points) across all species and tissue types of the first freezing-thawing cycle (Pearson correlation index values; *** show significance p of less than 0).
- Copyright: © 2025 by the author(s). Published by Maximum Academic Press, Fayetteville, GA. This article is an open access article distributed under Creative Commons Attribution License (CC BY 4.0), visit https://creativecommons.org/licenses/by/4.0/.
-
About this article
Cite this article
Švara A, Upchurch D, Goderis B, Colaers M, Deckers T, et al. 2025. Differential scanning calorimetry: a novel approach to study spring frost tolerance in pome tree floral tissues. Fruit Research 5: e013 doi: 10.48130/frures-0025-0003
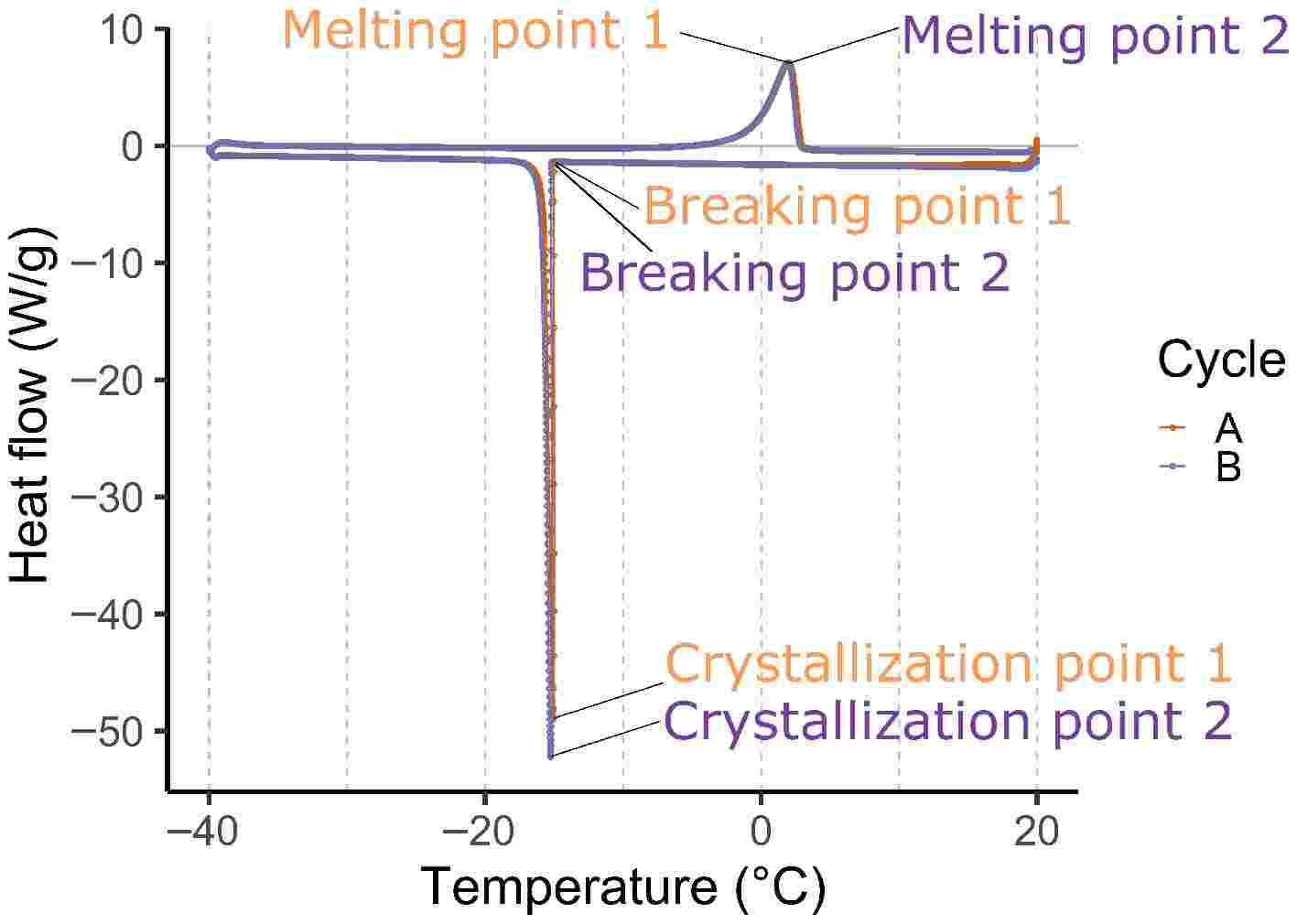