-
Combustible gas is a medium often encountered in the process of petrochemical, coal mining, storage and transportation. This media can burn and explode when it mixes with a certain concentration of oxygen or air and encounters an ignition source. Flame arresters are devices that can stop combustion in equipment and piping systems where flammable gases may be present[1], and the principle of flame arresting is mainly due to heat loss (heat transfer from the flame to the wall) or free radical quenching (reaction of flame radicals with the wall)[2−5]. According to this mechanism, many theoretical and experimental studies have been carried out for the propagation and quenching process of explosive flames in narrow channels.
Zamashchikov[6,7] found that the heat transfer between the flame and the channel wall may be the main factor determining the change in the shape of the symmetric flame front in the narrow channel by examining the flame propagation of propane-air and hydrogen-air mixtures in narrow channels with different pitches. As the spacing decreases, the extent of the flame in the narrow channel gradually decreases and the peak flame velocity shifts toward the combustion-rich region. Ding et al.[8] studied the flame propagation of premixed acetylene-air explosion under different spacing flat narrow channels. The results showed that the flame morphology shifted from tulip-like to mushroom-like in the channel with larger narrow channel spacing, which was due to the strengthening of wall heat dissipation to inhibit the formation of tulip-like flame. In contrast, the flame morphology in the narrow channels with smaller spacing forms a mushroom shape at first, and then the flame is gradually extinguished in the channel due to the enhanced wall heat dissipation. Borisov et al.[9] simulated the propagation of stoichiometric methane-air mixtures in the channels with different narrow channel spacing. The results show that flame extinction is achieved near the ignition source for small gap widths, while in wide gaps the flame propagates faster in the narrow channels due to the less influence of viscosity and heat loss. And the flame propagation speed was calculated as a function of gap width. Alexeev et al.[10] experimentally investigated propane flame propagation in different parallel narrow gaps. The results showed that in the gap width between 2.5 and 4.4 mm, the effect of heat loss on the normal flame velocity was significant and the effect of gap width on the cell structure was investigated and the flame cell characteristics were obtained for different gap widths. Li et al.[11] studied the flame development process and the variation law of the burst onset distance of ethylene/oxygen mixtures in different narrow gaps and obtained the variation of the burst onset distance with the variation trend of the gap height. The effect of gap height on the detonation onset distance gradually decreases with the increase of initial pressure. Huo et al.[12] obtained the variation law of flame propagation velocity with equivalent ratio for different narrow channel spacing, and the flame propagation velocity is highly sensitive to the change of spacing and is nonlinear. For different combustible gases there generally exists an optimal narrow channel spacing at which the flame propagation velocity is the fastest. The existence of the optimal spacing depends mainly on the flame surface tensile strength, and the optimal spacing size is influenced by the combination of wall heat dissipation and wall shear stress. Su et al.[13] experimentally studied the effect of equivalent ratio on the flame propagation characteristics of premixed propane/air explosion at different narrow channel spacing and observed that there are three flame front forms of flame propagation: smooth, wrinkled and fractured. The decrease of narrow channel spacing makes the flame more prone to crease phenomenon, and the flame propagation velocity increases and then decreases as the narrow channel spacing continues to decrease.
Some other scholars have conducted related studies on the change of channel spacing on the quenching characteristics of the explosion flame in the narrow channel. Gutkowski et al.[14] investigated the flame quenching characteristics of propane - air in narrow channel narrow channels with different pitches and found that after the chemical equivalent ratio propane-air flame propagation velocity in the narrow channel reached its peak, further increase in the narrow channel width would inhibit the flame propagation and make its flame propagation velocity decrease. Ciccarelli[15] pointed out experimentally that the quenching effect is closely related to the size of the gap. Sun[16] used a combination of experiments and simulations to investigate the firestopping performance of pipe flame arrestors. By analyzing the firestopping narrow channel spacing and thickness, it was concluded that the decrease in narrow channel spacing improves the firestopping performance while causing an increase in the pressure and pressure drop rate at the front end of the pipe. Wen et al.[17] conducted numerical simulations on the dynamic propagation and quenching characteristics of gas deflagration flame with different narrow channel spacing. The results show that the quenching distance is related to the narrow channel spacing, and the smaller the narrow channel spacing is, the quenching distance will be reduced and the flame will be more easily flame-retarded. Hu[18] experimentally studied the quenching characteristics of flame in narrow channel and gave the relationship between quenching length and narrow channel spacing based on the experimental results. Huang[19] used numerical simulation for different narrow channel size flame arresters and found that the narrow channel size is one of the main factors affecting the performance of flame arresters, and the increase of the narrow channel gap will make the flame arresting performance worse, but will promote the airflow in the flame arresters. Song[20] conducted an experimental study on the propagation and extinguishing process of premixed acetylene-air deflagration flame in a flat narrow channel. The results showed that under the same critical flame propagation velocity conditions, the extinguishing length value increased rapidly with the increase of narrow channel height, indicating that the narrow channel height has a significant effect on the propagation and extinguishing of premixed flames. Liu[21] studied the propagation and quenching characteristics of premixed methane-air flames at different narrow channel spacing by numerical simulation method, and found that the larger the narrow channel spacing, the larger the flame speed, the higher the flat narrow channel temperature, and the larger the quenching length of the flame inside the narrow channel; as the narrow channel spacing increased, the rise of the wall temperature was more obvious on the quenching length of the flame. Sun et al.[22] investigated the propagation and quenching of propane flames under different narrow channel sizes using numerical simulation methods. The results showed that the narrow channel size was positively correlated with the quenching length, and the narrow channel size was the main factor affecting the quenching length at higher initial flame velocity.
Scholars on the effect of narrow channel spacing on combustible gas explosion research focused on a single narrow channel, and mainly the narrow channel spacing on the impact of the explosion flame propagation, mostly focused on the simulation of the investigation, and relatively little experimental research on the explosion characteristics. And with the gradual reduction of the multi-layer narrow channel spacing, the flame morphology and explosion characteristics inside the narrow channel are affected very differently. Therefore, this study uses a laboratory-scale research platform to obtain the changes in flame propagation pattern and explosion characteristics of syngas explosion under different narrow channel spacing.
-
As shown in Fig. 1, the experimental setup mainly is made up of the explosion piping system, gas distribution system, ignition system, data acquisition system, synchronization control system, parallel narrow channel device and the corresponding auxiliary equipment.
The cross section of explosion pipes is 30 mm × 30 mm, which is made from 304 stainless steel. The explosion pipes include a acceleration pipe with 600 mm in length, a visualization pipe with 450 mm in length and a buffer pipe with 350 mm in length. The visualization pipe is equipped with a 450 mm × 30 mm quartz viewing window to capture the explosion flame. The parallel narrow channel device is installed in the middle of the visualization pipe, which is built by stainless steel plates with 290 mm × 30 mm × 1 mm in three dimensions respectively, as shown in Fig. 2. The gas distribution system mainly consists of gas cylinder, air compressor, mass flow controller and drying tube. The synthesis gas and air are dried through the drying tube and then passed into the pipeline through two mass flow controllers (range 5 and 10 L/min, accuracy ± 1.5%FS, maximum pressure 3 MPa). The data acquisition system mainly consists of a high-speed camera, sensors, a data acquisition board and a computer. The FASTCAM Mini UX100 high-speed camera is employed to capture the dynamic process of explosion flame at a rate of 8000 FPS. The explosion pressure and flame temperature are measured by two piezoresistive sensors (range 0−6 MPa, accuracy ± 0.5%, sampling frequency 200 kHz) and a thermocouple (WRe3-WRe25 type, measuring temperature range 0−2,300 °C), which are flush mounted on the parallel narrow channel. The data during the explosion is logged by the ART-PCIE9770 data acquisition card at a frequency of 200 kHz. The synchronization control system is employed to simultaneously trigger the high-pressure package igniter, the data acquisition card and the high-speed camera. The ignition position is 570 mm far away from the entrance of the narrow channel.
Experimental method
-
The premixed syngas (CO : H2 = 1:1) and air with stoichiometric ratio is used in experiments. Experiments are conducted at ambient temperature and pressure. Parallel narrow channels with three intervals D1 = 1.0 mm, D2 = 0.5 mm and D3 = 0.3 mm are employed in experiments to investigate the impact of channel spacing on the explosion characteristic in parallel narrow channels. Each experiment is repeated three times to eliminate error.
-
The flame propagation images with different narrow channel spacing were obtained using a high-speed camera. Figure 3 shows the flame propagation images at different narrow channel spacing.
The flame before the entrance of parallel narrow channels is different for different narrow channel spacing, which is normal finger-shaped flame at narrow channel spacing 1.0 mm, an upward single-headed finger-shaped flame at spacing 0.5 mm and a flat-headed finger-shaped flame at spacing 0.3 mm. This is because that the cross-sectional area of the parallel plate gradually increases with spacing decreasing, which leads to the explosion reflected wave produced by the parallel narrow channel plates becoming more and more intense. Therefore, the finger shape flame front gradually becomes flat under the influence of the reflected wave.
The flame in the parallel narrow channels inherits the flame front pattern at the front of the narrow channel entrance. When the flame enters the parallel narrow channels, it shows a finger-shaped flame at different narrow channel spacing. And then the flame shape gradually changed. At the narrow channel spacing 1.0 mm (as shown in Fig. 3a), the flame front gradually evolved from the normal finger shape at the entrance of the narrow channel to a slender single-headed finger shape. After propagating in the narrow channel for a period of time, the slender finger flame front gradually flattens (28.2 ms) and gradually forms a variant tulip flame (28.6 ms). Then the flame front continued to stretch at the exit of the narrow channel, turning into a variant tulip shape with a stretched upper end (29.6 ms).
At the narrow channel spacing of 0.5 mm, the single-headed finger flame gradually flattens after entering the narrow channel (29.9 ms), and then the upper flame inside the narrow channel stretches into the same upward single-headed finger flame as at the entrance. The same upper-end stretched variant tulip flame shape forms at the exit of the narrow channel at the time of 33.0 ms in Fig. 3b.
And at the channel spacing of 0.3 mm, the flame gradually changed from a flat-headed finger flame when it first enters the narrow channel to a finger flame stretched downward (34.0 ms), and keeps the finger shape propagating to the exit of the narrow channel.
As shown in Fig. 3, with the decrease of the narrow channel spacing, the flame following after the flame front within the narrow channel becomes dimmer and may even quench. On one hand, after the flame front passes through, the residual reaction gradually decreases, resulting in the heat generation of reaction decrease. On the other hand, as the narrow channel spacing decreases, the contact area between the flame and the channel walls increases, resulting in enhancing the heat exchange between the flame and the cold wall. As a result, the heat generated by explosion is insufficient to offset the heat absorbed by the cold wall, resulting in flame dimming or quenching inside the narrow channel.
Variation of flame front position and velocity in the narrow channel
-
Fig. 4 shows the flame front position and velocity change at different narrow channel spacing. It should be noted that the flame front position in Fig. 4 starts at the entrance of the parallel narrow channels and the time starts at the moment of the flame front entering the narrow channel. As the parallel narrow channel spacing decreasing, the time for the flame front passing throughout the narrow channel increases, which increases from 3.8 ms for channel spacing 1.0 mm to 4.9 ms for channel spacing 0.3 mm.
As shown in Fig. 4, after entering into the parallel narrow channels the propagation velocity of flame front increases to a peak value, which is because that combustion reaction in the narrow channels supplies continuous energy to flame acceleration. The maximum velocities of the flame front are 124.6 m/s, 100.2 and 93.8 m/s for the channel spacing of 1.0, 0.5 and 0.3 mm respectively. The maximum velocity decreases as channel spacing decreases. This is because the heat absorbed by channel wall increases as the channel spacing decreases.
The location of the flame velocity arriving at its peak is about the middle or rear of the channel, due to the reflected pressure by the end of buffer pipe. The duration of flame front accelerating to maximum flame velocity increases with decreasing narrow channel spacing. As shown in Fig. 3a, at the narrow channel spacing of 1.0 mm, the tulip flame is observed to form within the narrow channel, indicating an increase in the propagation speed of the flame front inside the narrow channel[23]. As a result, the flame front velocity for channel spacing 1.0 mm increases again.
The effect of narrow channel spacing on the explosion pressure
-
The explosion pressure variation curves at the front and rear ends inside the narrow channel under different narrow channel spacing are presented in Fig. 5. There is a duration of pressure starting to rise, which is called trise. The explosion pressure rises at a very fast rate from initial pressure to the maximum explosion pressure (Pmax), and then slowly declines. As shown in Fig. 5c, trise increases as the narrow channel spacing decreasing, which indicates the channel spacing has an effect on the propagation of pressure wave.
Fig. 5d shows the variation of Pmax at different narrow channel spacing. As the channel spacing decreases, Pmax decreases. And the maximum explosion pressure rise rate (dP/dt)max also increases as the narrow channel spacing increasing, as shown in Fig. 6. When the channel spacing decrease, the heat generated by explosion decreases and the heat absorbed by channel walls increases in the parallel narrow channels. As a result, the explosion in the channel weakens (as shown in Fig. 3), which leads to a lower Pmax.
Pmax in the back end of the narrow channel is larger than that in the front end, which is especially obvious for the narrow channel spacing 1.0 mm, as well as (dP/dt)max. This is because the wall heat dissipation for channel spacing 1.0 mm is less than that for channel spacing 0.3 mm. As a result, the explosion reaction inside the channel for spacing 1.0 mm is more intense than that for spacing 0.3 mm.
Effect of narrow channel spacing on explosion flame temperature
-
Fig. 7 shows the temperature and temperature rise rate for different channel spacing. There is a duration of temperature starting to rise, which is about 28 ms. The explosion flame temperature rises rapidly from initial value to the maximum explosion flame temperature (Tmax), and then declines with fluctuation. It is because the flame passes back into the narrow channels from the buffer pipe.
Fig. 7b shows the variation of the temperature rise rate at different narrow channel spacing. The temperature rise rate decreases as the narrow channel spacing decreases. And there is another peak due to the flame passing into the narrow channels backwards and forwards from the buffer pipe. As the narrow channel spacing decreasing, the maximum explosion flame temperature of the flame inside the narrow channel also gradually decreases, which is because the explosion weaken.
-
Experiments of premixed syngas-air explosion inside a parallel narrow channels are conducted. The flame propagation, explosion pressure and temperature inside the narrow channel are investigated to find out the effect of narrow channel spacing on the explosion.
(1) As the narrow channel spacing decreasing, the flame front shape before the entrance of narrow channel changes from a normal finger shape for channel spacing 1.0 mm to a flattened shape for channel spacing 0.3 mm. In the narrow channel, the flame inherits the flame front pattern at the front of the narrow channel entrance, and then develops. The tulip flame inside the narrow channel is observed for channel spacing 1 mm. While a variant tulip flame appears only at the end of the narrow channel for spacing 0.5 mm. With the decrease of the narrow channel spacing, the flame following after the flame front within the narrow channel becomes dimmer and may even quench, which is because the heat generated by explosion is insufficient to offset the heat absorbed by the cold wall. The flame inside the narrow channels accelerates and reaches a peak value at the middle or rear of the channel. The flame front velocity decreases as the channel spacing decreasing.
(2) As the narrow channel spacing decreasing, the maximum explosion pressure Pmax and the maximum explosion pressure rise rate (dP/dt)max decrease due to the explosion inside the channel being weakened by the channel walls. Pmax in the back end of the narrow channel is larger than that in the front end, as well as (dP/dt)max.
(3) The explosion flame temperature inside the narrow channels rises rapidly from initial value to the maximum explosion flame temperature (Tmax), and then declines with fluctuation due to the flame passing back into the narrow channels from the buffer pipe. The explosion flame temperature and explosion flame temperature rise rate decrease with decrease of channel spacing.
The authors are grateful for National Natural Science Foundation of China (No. 52074159 and No. 52004132), Key R & D programs (Social Development) in Jiangsu Province (No. BE2020710), Key National Natural Science Foundation of China (No. 51834007), Key Natural Science Foundation in Jiangsu Province (No. 18KJA620003) and Jiangsu Project Plan for Outstanding Talents Team in Six Research Fields (TD-XNYQC-002).
-
The authors declare that they have no conflict of interest. Zhirong Wang is the Editorial Board member of Emergency Management Science and Technology who was blinded from reviewing or making decisions on the manuscript. The article was subject to the journal's standard procedures, with peer-review handled independently of this Editorial Board members and his research groups.
- Copyright: © 2023 by the author(s). Published by Maximum Academic Press on behalf of Nanjing Tech University. This article is an open access article distributed under Creative Commons Attribution License (CC BY 4.0), visit https://creativecommons.org/licenses/by/4.0/.
-
About this article
Cite this article
Guo P, Xu C, Lu J, Wang Z, Chang X, et al. 2023. Study on the effect of channel spacing on premixed syngas-air explosion inside parallel narrow channels. Emergency Management Science and Technology 3:8 doi: 10.48130/EMST-2023-0008
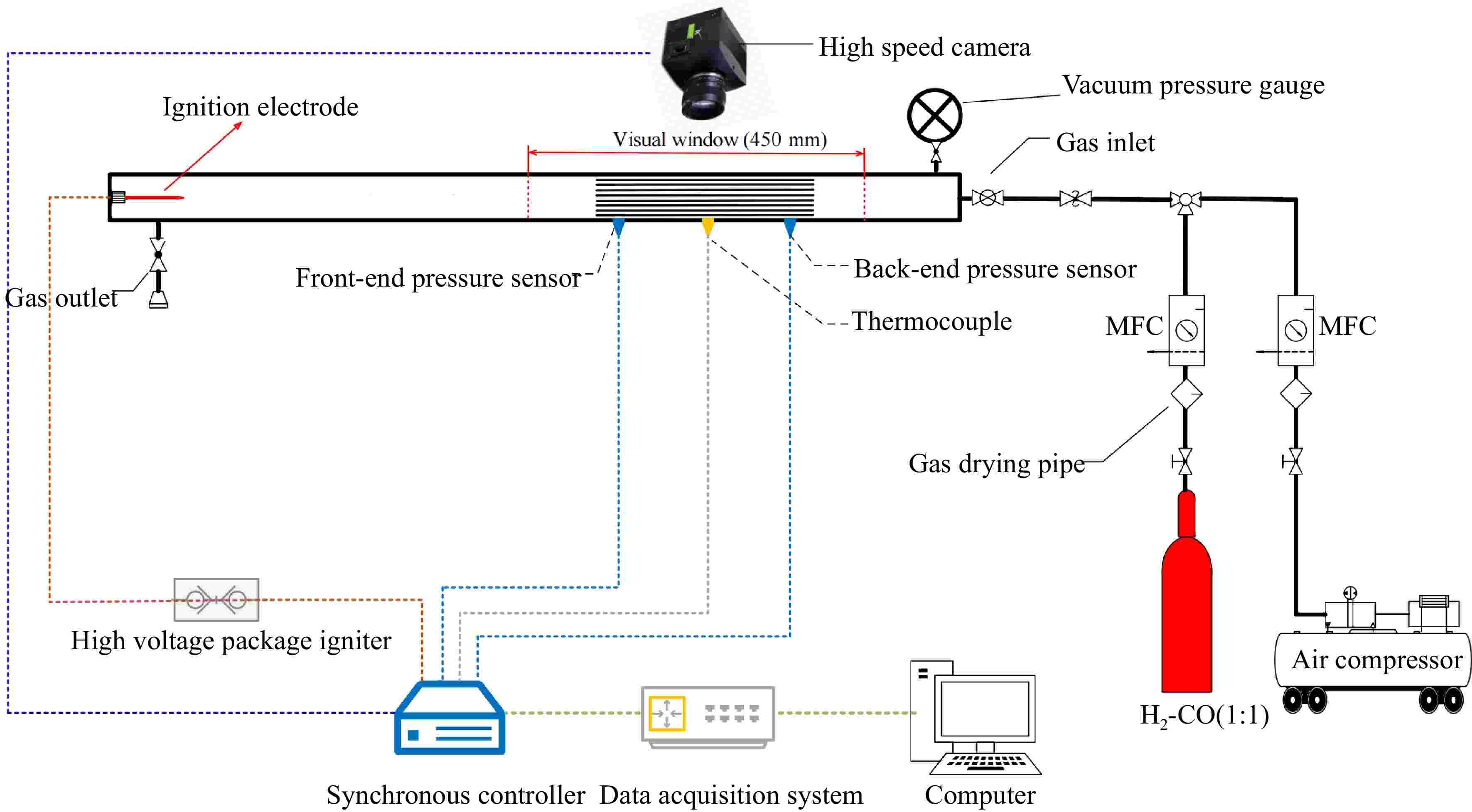