-
Strawberry is an important economic crop with delicious and nutritious fruit[1,2]. In north China, strawberry is usually cultivated in solar greenhouses with forcing cultivation during the winter and early spring season. Forcing cultivation has the advantages of breaking dormancy and prolonging the harvest time in strawberry. However, under this condition, low light intensities and short-days are the major obstacles that restrict strawberry growth and development. There are many reasons for this problem, including short sunshine time and frequent hazy weather in winter, covering for heat preservation, and reducing light transmittance after the surface of the shed film absorbs dust, etc. These conditions may lead to reduced photosynthesis and thus affect plant growth and development of strawberry. Therefore, it is crucial to solve the problem of insufficient light in strawberry production.
The artificial supplemental light source is an important cultivation technology for strawberry plants grown in greenhouses[3,4]. LED (Light Emitting Diode) was widely used in horticultural production in controlled environments due to the advantages of small size, relatively low heat release, great wavelength controllability, long lifetime, and low power consumption[5,6]. Different light wavelengths have different effects on strawberry plants' development and fruit quality[7,8]. To improve light-use efficiency in a greenhouse, more and more researchers pay attention to which corresponding light wavelengths are required for different growth and development processes of plants[9].
Red light is one of the most efficient light qualities for powering plants' photosynthesis[10,11], but monochromatic red light may cause abnormal phenotypes because of a lower photosynthetic rate in several crop plants, such as leaf curling and leaf thickness, etc[12,13]. Adding blue light can suppress these symptoms[12−14]. Other studies showed that red and blue light mixed wavelength is beneficial to enhance strawberry production and fruit quality[15,16]. However, the effective model of R/B light supplementation in strawberry cultivar 'Yanli' is poorly understood.
Strawberry cultivar 'Yanli' was released from Shenyang Agricultural University, China. The fruit has excellent flavor and aroma and the exterior is a regular conic shape and bright red. It is grown in different provinces of China, especially cultivated by forcing cultivation in solar greenhouses in northern China[17]. Therefore, it is necessary to study its effective cultivation practices. We established LED facilities to supplement the red/blue light (R/B = 4:1) for 'Yanli' before sunrise and after sunset in the solar greenhouse. The results showed that the photosynthetic efficiency, soluble solid content and yield were increased. In addition, we initially screened the candidate genes for improving photosynthesis and fruit quality in 'Yanli' strawberry under the condition of red/blue light supplementation by RNA-seq. These results will help to perfect the cultivation techniques for strawberry cultivar 'Yanli'.
-
'Yanli' (Fragaria × ananassa Duch.) plants were grown in the greenhouse at Shenyang Agricultural University (Liaoning province, China). Runner plants of 'Yanli' were planted in the greenhouse in early September every year. After 30 d, 20 of them were subjected to the condition of a mixture of red (R) and blue (B) light at 06:30−08:00 AM (before sunrise) and 15:50−19:00 PM (after sunset) daily. Supplemental light treatments were provided using LED lamps (North Brilliancy Technology Co., LTD, Shenzhen, China). These LED lamps were installed at 1.5 m above the strawberry plants. The photosynthetic photon flux density (PPFD) was measured by a quantum sensor (LI-250A, LI-COR, USA), and the PPFD ratio of red (3.9261 μmol·m–2·s–1) to blue (0.9716 μmol·m–2·s–1) to supplemental light (R/B) was 4:1. Plants without any supplemental light were used as control.
Measurements of growth and physiological traits
-
The height and crown diameter of strawberry plants were measured before reproductive growth. Plant height was measured from the ground to the highest blade. The crown diameter was the diameter of the leaf clusters. Primary fresh fruits were weighed. During the ripening stages of strawberry, the soluble solid content (SSC) of the strawberry juice was measured by a digital pocket refractometer ATAGO PAL-α (Atago Co. Ltd., Tokyo, Japan), and the firmness of fresh fruit was measured using a texture analyzer (GY-4, Handpi). Two opposite sites on the fruit shoulder were measured for each fruit, and the average firmness value of each fruit was recorded. Photosynthetic indexes, including net photosynthetic rate(Pn), stomata conductance (Gs), intercellular CO2 (Ci), and transpiration rate (Tr), were measured with a portable photosynthesis system (CIRAS-2, PP Systems, Massachusetts, USA) within the hours of 8:30−11:30 AM under sunny conditions. Third fully opened and new leaves from each plant were used for the photosynthetic indexes data. Twenty plants were measured for treatment and control, and three fruits were measured per plant.
RNA extraction and sequencing
-
After 50 d of irradiation, the fully opened and new leaves of treated strawberry plants and controls in three biological replicates were selected for sampling and frozen in liquid nitrogen. Total RNA was extracted by a modified CTAB method[18]. RNA concentration was measured using a NanoDrop 2000 (Thermo Fisher Scientific, Wilmington, DE, USA). cDNA library and high-throughput sequencing were conducted by Biomarker Technologies Co, LTD (Beijing, China). The Illumina HiSeq™ platform was performed. Reads from each library were de novo assembled separately. Gene expression levels were measured in the RNA-Seq analysis as fragments per kilobase per million mapped fragments (FPKM).
Statistical analysis
-
The data for the physiological parameters were analyzed using DPS 7.05 software. Significant differences between treatment and control were evaluated with Student's t-test, and the significance level was set at * p < 0.05 and ** p < 0.01, respectively.
-
Aiming to elucidate whether red/blue (R/B) light supplementation affects the growth and development of 'Yanli' strawberry, we compared the plants growing under R/B light supplementation and control conditions. We found that R/B light supplementation significantly increased the growth of strawberry plants in two years (Supplemental Fig. S1). During 2019−2020 and 2020−2021, the plant height of the strawberry under supplemental R/B lighting was 17% and 13% higher than that of the control, respectively (Table 1). Meanwhile, the crown diameter of the plants under light treatment was increased by 1.38-fold and 1.07-fold compared with that in the control during the two years, respectively (Table 1). To further explore the effect of supplemental R/B light on the photosynthesis of 'Yanli' strawberry, we measured the photosynthetic parameters of 'Yanli' strawberry plants during the flowering and fruit setting stage. The results showed that the Pn, Gs and Tr of strawberry plants under R/B light supplementation were 19%, 55% and 27% higher than those in the control, respectively (Fig. 1).
Table 1. Comparison of strawberry plant height and crown diameter between red/blue light supplementation and control.
Year Light treatment Plant height (cm) Crown diameter (cm) 2019−2020 Control 17.11 22.42 RB 19.96** 30.86** 2020−2021 Control 17.22 24.23 RB 19.44* 26.04** Figure 1.
Effect of R/B light supplementation on photosynthetic parameters of strawberry cultivar 'Yanli' cultured in solar greenhouse. (a)−(d) net photosynthetic rate (Pn), stomata conductance (Gs), intercellular CO2 (Ci), and transpiration rate (Tr). Vertical bars represent the SD. Statistical significance was measured using Student's t-test. (n = 20, * p < 0.05, ** p < 0.01).
Effects of supplemental R/B light on strawberry yield and fruit quality
-
To investigate whether supplemental R/B light affected strawberry yield and fruit quality, we analyzed strawberry primary fruit fresh weight, total fruit weight/plant, SSC, and the fruit firmness under supplemental R/B light and control. As shown in Fig. 2a, the R/B light supplementation led to a remarkable improvement (18% and 24%) in the strawberry primary fruit fresh weight during the stage of full ripeness compared with that in control conditions during the years 2019−2020 and 2020−2021. And total fruit weight/plant under supplemental R/B light was 27% and 33% higher than that of the control during two consecutive years, respectively (Fig. 2b). Under R/B light supplementation, strawberry fruit SSC was increased by 1.21-fold and 1.05-fold compared with that in the control during the two years, respectively (Fig. 2c). Similarly, fruit firmness was also increased by 1.18-fold and 1.06-fold compared with that in the control for two years, respectively (Fig. 2d). These results suggested that supplemental R/B light could improve 'Yanli' strawberry yield and fruit quality.
Figure 2.
Effect of R/B light supplementation on production and fruit quality of strawberry cultivar 'Yanli' cultured in solar greenhouse. (a) Primary fruit fresh weight, (b) total fruit weight/plant, (c) soluble solid content and (d) firmness of the 'Yanli' fruit under R/B light supplementation and control. Vertical bars represent SD. Statistical significance was measured using Student's t-test. (n = 20, * p < 0.05, ** p < 0.01).
Transcriptome sequencing
-
To study the effect of supplemental R/B light on 'Yanli' strawberry plants, total RNA from six samples (CK-1, CK-2, CK-3 and RB-1, RB-2, RB-3) were used for RNA-seq. The treatment and control group respectively contain three biological repeat samples. After filtering dirty tags from the raw data, a total of 38.36 Gb of clean data were obtained, with an average of 6.21 Gb of clean reads per sample. Of the clean reads, the average GC content is approximately 46.96%, and the Q30 percentage was over 93.05%. The percentage of clean reads mapped to the unigene database ranged from 79.07% to 80.42% (Table 2).
Table 2. Summary of transcriptome data.
Sample Clean read number Clean base number GC contenta % ≥ Q30b Mapped reads Mapped ratio CK-1 21,222,192 6,353,022,700 46.98% 93.13% 16,779,949 79.07% CK-2 23,479,291 7,032,924,424 47.23% 93.04% 18,653,551 79.45% CK-3 20,727,367 6,210,109,126 47.07% 93.09% 16,668,439 80.42% RB-1 20,756,139 6,218,258,998 46.63% 93.07% 16,594,034 79.95% RB-2 20,842,520 6,243,435,538 46.83% 92.85% 16,552,377 79.42% RB-3 21,022,752 6,297,302,712 46.99% 93.09% 16,753,654 79.69% a GC Content: the percentage of G and C bases in the total bases in clean reads. b % ≥ Q30: the percentages of clean reads with Phred qualities scores over 30. The FPKM values were used to analyze the gene expression levels in the RNA-seq analysis. R (Pearson correlation coefficient) was used as the evaluation index of correlation between each sample, the results of Fig. 3a showed that there was a strong correlation between the three biological replicates from each group. 1.5 fold change and P value less than 0.05 were used to define differentially expressed genes (DEGs). A total of 165 DEGs were identified, among which 76 were up-regulated and 89 were down-regulated by supplementing R/B light, respectively (Supplemental Table S1, Fig. 3b).
Figure 3.
Overview of the transcriptome sequencing under supplemental R/B lighting in strawberry leaves. (a) Pearson correlation between samples analysis. (b) Number of up- and down-regulated expressed genes. CK-1, CK-2, CK-3 are controls, RB-1, RB-2, and RB-3 are the experimental groups treated with supplemental R/B lighting.
GO and KEGG pathway enrichment
-
GO (Gene ontology) functional classes showed that the putative function of DEGs. The enriched genes were classified into three major categories, including Biological Processes (BP), Cellular Components (CC), and Molecular Functions (MF). Metabolic process, cellular process and single-organism process were enriched mainly in biological processes. Among CC, cell, cell part, membrane, membrane part and organelle were the main terms. Molecular functions such as binding, catalytic activity and transporter activity were mainly enriched (Fig. 4). And TopGO was used to analyze the function enrichment of DEGs, including in the oxidation-reduction process (GO:0055114, KS = 9.5e-10), photosynthesis (GO:0015979, 6.7e-05), chloroplast thylakoid membrane (GO:0009535, 0.00014; Supplemental Table S2). The annotated unigenes were classified into 14 COG (Cluster of Orthologous Groups of proteins) categories (Fig. 5). And the largest group is 'carbohydrate transport and metabolism'.
Figure 4.
Gene ontology (GO) enrichment analysis of differentially expressed genes in strawberry leaves by supplementing R/B light.
In addition, KEGG (Kyoto Encyclopedia of Genes and Genomes) annotation was used to analyze the functional enrichment of DEGs. There were 18 significantly enriched KEGG pathways (Fig. 6). In the comparison of the control and R/B light supplementation groups, the identified DEGs were mainly enriched in plant hormone signaling transduction, base excision repair, circadian rhythm-plant, and alpha-Linolenic acid metabolism.
Figure 6.
KEGG enrichment analysis of DEGs regulated by supplementing R/B light in strawberry leaves.
Transcriptomic analysis of some DEGs in response to red/blue light supplementation
-
Transcription factors (TFs) play an important role in plant response to changing environments[19]. In 'Yanli' leaves, the expressions of a total of 13 TF genes were significantly affected by supplementing R/B light. These TF genes belong to different families (NAC, PRR, MYB, bZIP, ZAT, CDF, CO, bHLH, BBX and PAR) (Table 3). The gene families including PRR, MYB, CDF and CO play an important role in the regulation of the circadian clock[20−23]. bHLH, BBX and PAR participate in the light signal transduction[24−26]. We found that PRR95 (PRR family), LHY (MYB family) and CDF3 (CDF family) were up-regulated, while CO16 (CO family), bHLH63 (Arabidopsis thaliana CIB1 homologous gene, bHLH family), BBX21 (BBX family) and PAR1 (PAR family) were down-regulated in 'Yanli' leaves during supplementing R/B light. In addition, we found that the SIGE regulating the expression of the chloroplast genes in the light-signaling pathway was upregulated (Supplemental Table S1). The results indicated that these TFs and SIGE maybe participate in the photosynthesis process of 'Yanli' during R/B light supplementation.
Table 3. List of differentially expressed TF genes.
Gene family TF name Annotation function logFC Regulation NAC NAC72 NAC domain-containing protein 72-like 0.931857 Up PRR PRR95 Two-component response regulator-like PRR95 0.893515 Up MYB LHY Protein LHY-like 0.657352 Up bZIP TRAB1 bZIP transcription factor TRAB1 0.651141 Up ZAT ZAT8 Zinc finger protein ZAT8-like 0.628283 Up CDF CDF3 Cyclic dof factor 3 0.641524 Up CO CO16 Zinc finger protein CONSTANS-LIKE 16 −0.66291 Down bZIP bZIP34 Basic leucine zipper 34 −1.07449 Down bZIP bZIP61 Basic leucine zipper 61-like −0.9109 Down bHLH bHLH63 Transcription factor bHLH63 −0.66341 Down BBX BBX21 B-box zinc finger protein 21 −0.67538 Down PAR PAR1 Transcription factor PAR1 −0.61181 Down MYB RAD Transcription factor RADIALIS-like −0.61324 Down -
Light is one of the most vital environmental factors for plant growth and development. Too much or too little light can have adverse effects on some horticultural crops[27−29]. In winter, the cultivation of horticultural crops in solar greenhouses is widely used to meet people's nutritional needs, in northern China[30]. In addition to insufficient light time in northern winter, low PPFD under greenhouse conditions is also one of the important factors restricting the yields and quality of horticultural crops[28,31]. More and more studies found that combined red and blue light supplementation have a widely positive effect on the process of growth and development, and fruit quality for horticultural plants[32−34]. There are also individual differences between different red and blue ratios, different horticultural plants and different varieties[15,32,35]. The supplementation of red/blue (4:1) light for 3 h every day improved the growth of pepper seedlings in solar greenhouse[36]. Tomatoes grown under red/blue light (ratio = 3:1) have optimum growth[37]. In order to explore suitable cultivation measures for the 'Yanli' strawberry, and LED red and blue light (ratio = 4:1) was supplemented without changing other conditions in the solar greenhouse. Combined with transcriptome analysis and phenotypic identification, we found that supplementation of red and blue light could improve photosynthesis, yield and soluble solid content in strawberry cultivar 'Yanli'.
For plant morphology and physiological indicators, we observed that the plant height, crown diameter and photosynthesis were significantly increased in 'Yanli' strawberry under R/B light supplementation before sunrise and after sunset every day. Previous studies have also shown that red and blue light can improve plant growth and photosynthesis. Strawberry quality and production were improved by promoting the stomatal opening and accumulation of the photosynthetic products under the condition of supplementary morning lighting with blue light[5]. In addition, we compared the transcriptomes of the leaves of 'Yanli' strawberry plants under supplemental red and blue light and control.
Many TFs regulate photosynthesis gene expression and response to changing environmental conditions[30,38]. We found several different expression TF genes in 'Yanli' leaves between supplementing R/B light and control. PRR95, LHY and CDF3 were up-regulated, bHLH63, BBX21 and PAR1 were down-regulated. Among them, PRR and LHY are the downstream photoreceptors and the key component of the circadian clock[39]. Photosynthesis is regulated by the circadian rhythm, and it is very important that the circadian clock coordinates photosynthesis to improve the efficiency of light-energy capture and carbon fixation[20]. In Jatropha curcas, CDFs (cycling dof factors) responded to the photoperiod[23]. CDFs also play an important role in improving photosynthetic efficiency, coordinating carbon/nitrogen balance and promoting plant growth[40]. For CIB1 (CRYPTOCHROME-INTERACTING BHLH 1), FvebHLH63 homologous gene, previous studies show that CIB1 negatively regulates the photosynthetic rate in soybean[41]. BBXs play an important role in photomorphosis. BBXs and HY5 are components that control a variety of light-regulated genes expression[42−44]. PAR regulated hypocotyl length under simulated shade in Arabidopsis[45]. The function of PAR1 in strawberry needs to be further studied. Besides, RNA polymerase sigma factor gene SIGE (a homolog of SIG5 in Arabidopsis) was upregulated by supplementing R/B light. SIG5 is mainly induced by red light and blue light to participate in photosynthesis[46,47]. SIG5 regulates the chloroplast genes psbD and psbA (PSII core proteins) in chloroplasts at the post-transcriptional level[48,49]. In the present study, transcriptome analysis indicated PRR95, LHY, CDF3, CO16, bHLH63, BBX21, PAR1 and SIGE exhibited different expressions between supplemental R/B light and control in 'Yanli'. We speculate that these eight differentially expressed genes maybe participate in the photosynthesis process of 'Yanli' during R/B light supplementation.
For fruit quality and yield, previous studies have shown that tomato fruit quality was improved by increased photosynthetic efficiency under red and blue LED (red : blue = 8:2) supplementation in the greenhouse[50]. In the condition of an artificial climate chamber, supplementing R/B light in the morning promoted the accumulation of health-promoting substances in the tomato fruits, such as vitamin C, organic acids, carotenoids, etc. While the content of sugars, flavonoids, and aromatic substances in tomato fruits were significantly increased by supplementing R/B light at night[51]. In addition, the photosynthetic activity, and fruit productivity were increased in strawberry plants under different ratios of red and blue LED light[52,53]. In this study, we found that the SSC content, primary fruit weight, and fruit weight per plant were increased by R/B light supplementation in 'Yanli' strawberry. At the same time, transcriptome analysis found that the expression of genes related to sucrose transport (sugar transporter: SWEET9-like, c50514.graph_c0) and amylolysis (beta-amylase: BAM1, c61305.graph_c0) were up-regulated (Supplemental Table S1). This may be related to strawberry fruit quality. Some studies showed that there were no effects on the strawberry fruit firmness after monochromatic red or blue light treatment[54,55]. However, our studies found that the strawberry fruit firmness was increased by supplementing R/B light. This is probably a consequence of different strawberry varieties or different cultivation environments. Next, we will further explore the differentially expressed genes in 'Yanli' between R/B light supplementation and control.
For the most suitable ratio of supplementary R/B light and the photoperiod for strawberry cultivars 'Yanli', we are investigating the growth and development of 'Yanli' strawberry plants in the artificial climate culture chamber under different R/B light ratio and photoperiod, so as to be applied to 'Yanli' cultivation and production more accurately. Different red and blue ratios have different effects on different species or different traits of the same species[35,37]. In this study, we found that the growth, photosynthesis, yield and fruit quality of 'Yanli' strawberries were improved under R/B light (red : blue = 4:1) supplementary in solar greenhouses of northern China. The cost for LED lights is about $600 per thousand square meters, and the LED lights could be used for 3−5 years. The economic benefits far outweigh the costs of LED lights. Therefore, these studies provide evidence for establishing the effective cultivation measures of 'Yanli' strawberry. The internal molecular mechanism still needs to be further studied.
This work was supported by National Natural Science Foundation of China (Grant No. 32130092, 32102350, 31872072) and LiaoNing Revitalization Talents Program (No. XLYC1902069).
-
Zhihong Zhang is the Editorial Board member of the journal Fruit Research. He was blinded from reviewing or making decisions on the manuscript. The article was subject to the journal's standard procedures, with peer-review handled independently of this Editorial Board member and his research group.
- Supplemental Table S1 All DEGs.
- Supplemental Table S2 Top GO.
- Supplemental Fig. S1 Phenotype of strawberry cultivar ‘Yanli’ under supplemental R/B lighting (B) and control (A).
- Copyright: © 2023 by the author(s). Published by Maximum Academic Press, Fayetteville, GA. This article is an open access article distributed under Creative Commons Attribution License (CC BY 4.0), visit https://creativecommons.org/licenses/by/4.0/.
-
About this article
Cite this article
Wang Y, Tang X, Wang B, Dai H, Zhang Z. 2023. Positive effect of red/blue light supplementation on the photosynthetic capacity and fruit quality of 'Yanli' strawberry. Fruit Research 3:4 doi: 10.48130/FruRes-2023-0004
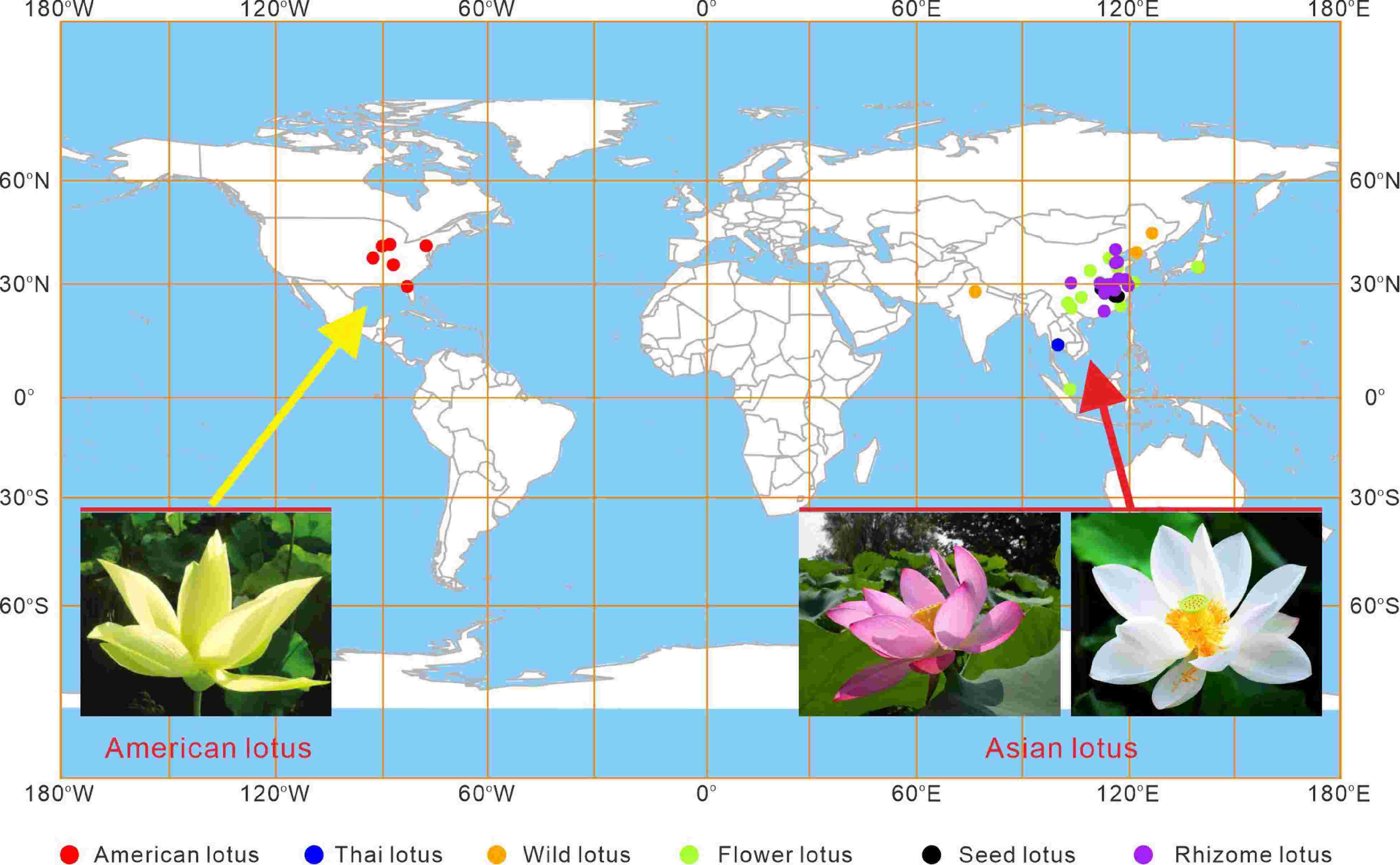