-
Coffee is among the most widely consumed pharmacologically active beverage world wide and its consumption has become part of everyday life[1]. Many coffee-producing countries in the coffee belt are benefiting from this agricultural commodity because their locations support the ideal growth and production of coffee, serving as a major source of income[2]. In particular, the Philippines is an ideal place to grow quality coffee of different types. However, despite the 2.1% increase in consumption, the local coffee production has been decreasing by 3.5% per year for the last 10 years[3].
Coffee cultivation is usually associated with agri-chemical inputs, such as fertilizers and pesticides, to increase soil fertility and crop growth. However, overuse of chemical fertilizers causes soil nutrient imbalance and environmental contamination[4]. Soil acidification due to unabsorbed chemical fertilizers may lead to plant toxicity, resulting in growth deterioration and low yield of crops. In addition, chemical fertilizers are expensive and may be a burden for smallholder coffee farmers. Sustainable strategies in agricultural farming are being introduced to combat such threats, such as the exploitation of beneficial microbes as a biofertilizer[5]. Rhizospheric microorganisms are being characterized to explore their potential role in food safety and sustainable crop production.
Rhizobacteria with plant growth promoting attributes and biocontrol activities are ubiquitous and highly abundant in the plant rhizosphere, which primarily colonize the roots and promote plant growth. They can act as biocontrol agent and their effects can occur via local antagonism to soil-borne pathogens or by induction of plant systemic resistance against pathogens[6]. Plant growth promotion can be attributed to their ability to produce phytohormones (e.g., indole acetic acid, cytokinins, and gibberellins), fix atmospheric nitrogen, solubilize phosphate, and mineralize organic substances[7−9].
This study focused on the isolation, characterization, and identification of rhizobacteria with phosphate solubilization ability from the coffee rhizosphere and we evaluated their biocontrol activities and tolerance to different abiotic stresses. Their potential use as a biofertilizer will provide new insights in coffee cultivation and production in the Philippines.
-
Soil samples were randomly collected at 35-cm soil depth along the roots of Coffea liberica (Liberica) and Coffea canephora (Robusta) trees at the Coffee Genebank of National Coffee Research, Development and Extension Center (NCRDEC) in Cavite State University. A total of three 100 g of rhizospheric soil sub-samples from each coffee tree were pooled to make one composite sample per tree. A portion of the collected samples were immediately processed for microbiological analysis and the rest were air-dried at room temperature for soil physicochemical analysis.
Soil physicochemical analysis
-
Dried soil samples were sent to the Agricultural System Institute, University of the Philippines Los Baños, for the analysis of pH, moisture content, and organic matter content. The pH of the soil was found to be at 5.2 and 5.7, moisture content was 41.6% and 42.3%, and organic matter content was about 2.88% and 3.28% in the Liberica and Robusta coffee rhizosphere, respectively.
Isolation of coffee rhizobacteria
-
We selectively isolated rhizobacteria with phosphate solubilization activity on tricalcium phosphate medium. Briefly, a total of 10 g of fresh composite soil samples were mixed in 90 mL sterile distilled water and was serially diluted to the 106 dilution. One mL from each dilution was spread-plated on Pikovskaya's agar medium, containing 0.5 g·L−1 yeast extract, 10.0 g·L−1 dextrose, 5.0 g·L−1 tricalcium phosphate, 0.5 g·L−1 ammonium sulphate, 0.2 g·L−1 potassium chloride, 0.1 g·L−1 magnesium sulphate, 0.0001 g·L−1 manganese sulphate, 0.0001 g·L−1 ferrous sulphate, and 15.0 g·L−1 agar. Agar plates were incubated at 30 °C for 5 d and the clear zone around colonies were observed as indication of phosphate solubilization. The colonies with a clear zone were purified on Nutrient Agar (NA) plates. The colony characteristics of the isolates, such as elevation, margin, shape, and color were recorded. In addition, the purity of the cultures was verified using Gram stain reaction, where bacterial shapes, arrangement, and Gram reaction were also recorded. Pure cultures were then stored at 4 °C for further analysis.
The phosphate solubilization index of rhizobacteria was measured, following the spot-plating method[10]. Initial numbers of cells were adjusted to 0.5 McFarland standard that is approximately 1.5 × 108 CFU·mL−1, this was used in all experimental assays of the study. A total of 4 μL 24-h bacterial suspension grown in Nutrient Broth (NB) was spot inoculated on Pikovskaya's agar plates containing tricalcium phosphate and incubated at 30 °C for 5 d. The colony and clear zone diameters (mm) were measured, then the solubilization index was calculated by dividing the sum of colony diameter and clear zone over the colony diameter.
Biocontrol activities of rhizobacteria
Hydrogen cyanide (HCN) production
-
All isolates were grown in a 5-mL glycine-supplemented NB medium. Initially, a Whatman filter paper was saturated with picric acid solution, containing 2% sodium carbonate and 0.5% picric acid, and was immediately placed at the inner top of the screw cap tubes. Tubes were sealed with parafilm and incubated with shaking for 4 d at room temperature. A color change from yellow to reddish brown in the filter paper indicate a positive HCN production.
Amylase activity
-
The bacterial inoculum was spot inoculated, in triplicates, on starch agar medium (containing 0.5 g·L−1 peptone, 3 g·L−1 beef extract, 0.5 g·L−1 NaCl, 1% starch powder, and 12 g·L−1 agar) and incubated for 48 h at 30 °C. The plates were then flooded with iodine solution for 1 min and then drained off. The appearance of a clear zone around colonies indicate a positive for amylase activity.
Protease activity
-
The protease activity of the isolates was screened using milk agar medium (containing 5 g·L−1 peptone, 3 g·L−1 yeast extract, 100 mL·L−1 UHT non-fat milk and 12 g·L−1 agar). A total of 4 μL fresh inoculum was spot inoculated on the medium, in triplicate, and incubated for 48 h at 30 °C. A clear zone around the colonies indicates a positive protease activity.
Pectinase activity
-
Pectinase activity of isolates was screened using the pectin agar medium (containing 0.5 g·L−1 peptone, 0.3 g·L−1 beef extract, 0.5 g·L−1 NaCl, 4 g·L−1 citrus pectin, and 12 g·L−1 agar). Spot inoculation was similarly used but incubation was extended to 96 h. A 50 mM potassium iodide-iodine solution was then flooded on the surface of the agar plates. A positive result was indicated by the appearance of a clear zone around colonies.
Abiotic stress tolerance of rhizobacteria
-
The ability of the isolates to tolerate abiotic stresses, such as acidity, salinity, and heavy metal contents (i.e., lead, copper, and manganese) was screened. Briefly, the isolates were subjected to varying pH level, namely 4, 5, 7, 9 and 11. Uniform initial number of cells were grown in NB for 24 h with shaking and bacterial suspension and spot inoculated on NA plates. Also, NA medium was supplemented with varying sodium chloride concentrations (i.e., 1%, 3%, 5%, 7%, 9%, and 11%) and was used to screen for salt tolerance of the isolates. Lastly, the ability of the isolates to tolerate heavy metals such as lead, copper, and manganese was screened using NA medium supplemented with varying concentrations (i.e., 100, 150, 200, 400, 800, and 1,600 ppm) of lead acetate (Pb(C2H3O2)2), copper sulphate (CuSO4), and manganese sulphate (MnSO4), respectively. Growth was observed after 48 h at room temperature, indicating resistance/tolerance to varying levels of pH, salt, and heavy metals.
Molecular identification of rhizobacteria
-
A total of six isolates were subjected to DNA extraction using Vivantis GF-1 Bacterial DNA Extraction Kit, following the manufacturer's protocol. The quality of the isolated genomic DNA was verified in 0.8% agarose gel (dissolved in 0.5X TAE buffer), following gel electrophoresis (Mupid One) and gel documentation (Vilber Lourmat). The purity and concentration of DNA were quantified using a NanoDrop 2000c UV-Vis Spectrophotometer (Thermo Scientific™).
Genomic DNA samples were subjected to polymerase chain reaction (PCR) amplification using universal primers to target the 16S rRNA gene, which are 27F (5'-AGAGTTTGATCCTGGCTCAG-3') and 1492R (5'-GGTTACCTTGTTACGACTT-3'). PCR was performed in a 50-μL reaction containing 1X Taq Master Mix (Vivantis), 2 mM MgCl2, 0.2 μM each of 27F and 1492R primers, and 100 ng of DNA template. A final volume of 50 μL was adjusted with molecular grade water. PCR reactions were performed using MiniAmp™ Plus Thermal Cycler (Applied Biosystems™) with the following conditions: initial denaturation step at 95 °C for 5 min, followed by 30 cycles of 94 °C for 30 s, annealing at 55 °C for 30 s, and 72 °C for 1 min, with a final extension step of 72 °C for 10 min. The PCR products were ran using 1.2% agarose gel (stained with GelRed® nucleic acid dye) under 100 V for 35 min and verified under UV transilluminator.
The PCR products were sent to Apical Scientific Sdn Bhd (Selangor, Malaysia) for Sanger sequencing. The electropherogram of the sequences was inspected and verified using Sequence Analyzer of MEGAX software. Homologous partial 16S rRNA gene sequences with > 97% similarity were mined in the GenBank database using BLAST algorithm. In MEGAX, ClustalW was used to perform multiple sequence alignment of the unknown and homologous nucleotide sequences. Phylogenetic trees were constructed using Neighbor joining method with 1,000 replicated bootstrap values. The identity of the rhizobacterial isolates was verified based on the clustering of target sequence with the closest annotated sequence (type strain).
-
A total of six rhizobacteria with phosphate solubilization activity (Fig. 1a) were recovered and were subjected to morphological characterization. Two isolates were Gram positive and four were Gram negative, by which all are rod-shaped cells. Colony shapes vary from irregular to circular; elevation is either flat, raised, convex, and crateriform; margin varies from being entire, serrate, undulate, and curled; and the color is yellow, cream, or white (Table 1). The solubilization index (SI) ranges 2.5 to 3.5 mm. Isolate PCL 2.1 and PCR 1.1 obtained the highest phosphate SI of 3.5 mm, whereas isolate PCL 1.3 obtained the lowest (2.5 mm) (Table 2).
Figure 1.
Representatives of rhizobacteria (a) capable of phosphate solubilization as indicated by the clear zone around the colony (arrow) and (b) not capable of phosphate solubilization grown on Pikovskaya's agar medium for 5 d at 30 °C.
Table 1. Morphological characteristics of rhizobacteria from the coffee rhizosphere.
Rhizosphere source Isolate Colony shape Colony elevation Colony margin Colony color Gram reaction Cell shape Liberica PCL 1.2 Irregular Raised Entire White Negative Rods Liberica PCL 2.1 Circular Flat Serrate White Positive Rods Liberica PCL 2.3 Irregular Convex Entire White Negative Rods Robusta PCR 1.1 Circular Raised Entire Yellow Negative Rods Robusta PCR 1.3 Irregular Crateriform Undulate Cream Positive Rods Robusta PCR 1.5 Circular Flat Curled White Negative Rods Table 2. Six rhizobacterial isolates showing phosphate solubilization, biocontrol activities, and abiotic stress tolerance.
Isolate Phosphate
solubilization
index (mm)Biocontrol activity Abiotic stress tolerance HCN production Amylase Protease Pectinase NaCl (%) pH MnSO4 (ppm) CuSO4 (ppm) Pb(C2H3O2)2 (ppm) PCL 1.2 3.1 ± 0.5 − − − − 7 4 – 11 1,600 400 800 PCL 2.1 3.5 ± 0.5 − + − − 7 4 – 11 1,600 400 800 PCL 2.3 3.1 ± 0.5 − − − − 3 4 – 11 1,600 400 800 PCR 1.1 3.5 ± 0.5 − − + − 3 4 – 11 1,600 800 800 PCR 1.3 2.5 ± 0.5 + + + − 5 4 – 11 800 400 800 PCR 1.5 2.8 ± 0.5 − + + + 3 4 – 11 1,600 400 800 HCN, hydrogen cyanide; NaCl, sodium chloride; MnSO4, manganese sulphate; CuSO4, copper sulphate; Pb(C2H3O2)2, lead acetate. Biocontrol activities of rhizobacteria
-
Among the six rhizobacterial isolates, only PCR 1.3 produced HCN. For the hydrolytic enzyme production, PCL 2.1 produced amylase, PCR 1.1 produced protease, PCR 1.3 produced amylase and protease, and PCR 1.5 produced amylase, protease, and pectinase (Table 2).
Abiotic stress tolerance of rhizobacteria
-
For pH tolerance, all isolates were tolerant to a wide range of pH (4 to 11). For salt tolerance, PCL 1.2 and PCL 2.1 were tolerant to 7% NaCl, while the lowest tolerance was observed at 3% NaCl. For heavy metal tolerance, all rhizobacteria tolerated 1600 ppm of MnSO4, except PCR 1.3 that only tolerated 800 ppm. PCR 1.1 was able to tolerate up to 800 ppm of CuSO4, while the rest were only tolerant to 400 ppm. Meanwhile, all of them were tolerant to 800 ppm of Pb(C2H3O2)2 (Table 2).
Molecular identity of rhizobacteria
-
Based on the 16S rRNA gene analysis of six promising rhizobacterial isolates, PCL 1.2, PCL 2.1, PCL 2.3, PCR 1.1, PCR 1.3, and PCR 1.5 were identified as Pantoea rwandensis (97.44%), Bacillus pseudomycoides (97.23%), Pantoea sp. (93.62%), Bukholderia cepacia (97.17%), Bacillus sanguinis (99.51%), and Bukholderia sp. (86.66%), respectively (Table 3). Based on the phylogenetic tree analysis, each of the unknown sequence clustered to its closest neighbor (type strain) with bootstrap values (Fig. 2).
Table 3. Molecular identity of rhizobacteria isolates from coffee rhizosphere.
Isolate Identity Closest neighbor (type strain) Similarity (%) Accession no. PCL 1.2 Pantoea rwandensis Pantoea rwandensis strain LMG 26275 97.44 NR_118121.1 PCL 2.1 Bacillus pseudomycoides Bacillus pseudomycoides 97.23 NR_114422.1 PCL 2.3 Pantoea sp. Pantoea agglomerans strain JCM1236 93.62 NR_111998.1 PCR 1.1 Burkholderia cepacia Burkholderia cepacia ATCC 25416 strain LMG 1222 97.17 NR_114491.1 PCR 1.3 Bacillus sanguinis Bacillus sanguinis strain BML-BC004 99.51 NR_175555.1 PCR 1.5 Burkholderia sp. Burkholderia pseudomallei strain ATCC 23343 86.66 NR_043553.1 -
Rhizosphere is a nutrient-rich region consisting of a wide variety of microorganisms living in the small area of soil that surrounds and is associated with plant roots. The organisms in the rhizosphere microbiome can have a significant influence on the development, nutrition, and health of plants[11]. Rod-shaped bacteria are commonly found in the rhizosphere and known to have plant growth-promoting attributes, including those species that belong to the genera Bacillus[6], Burkholderia[12], and Pantoea[13], which were isolated and characterized in our study.
The microbes in the rhizosphere play key roles in nutrient acquisition and assimilation, improved soil texture, secreting, and modulating extracellular molecules such as hormones, secondary metabolites, antibiotics, and various signal compounds, all leading to enhancement of plant growth[14]. Our study initially screened phosphate solubilizing bacteria, because their activity is essential to address plant phosphate requirements of various plants. These bacteria may provide the plants with available phosphorus from sources that would otherwise be scarce due to a wide range of mechanisms. They can also contribute to the natural biogeochemical cycle of nutrients in the rhizosphere[10,15]. Besides, inoculation of phosphate-solubilizing bacteria species has reportedly improved phosphorus absorption and grain production of Triticum aestivum[16] and it significantly facilitated the growth of Vitis vinifera under greenhouse conditions[17]. Besides, the indigenous bacteria with phosphate solubilization activity obtained from coffee rhizosphere were able to stimulate the growth of the Arabica coffee seedlings under nursery conditions[18]. In addition, phosphate solubilizing microbes generally improved the growth and nutrient uptake of Robusta coffee grown in phosphorus deficient soil[19]. The ability of the rhizobacterial isolates to solubilize inorganic phosphate indicates their potential role to improve the growth of crops. It is recommended to evaluate the amount of phosphorus that these rhizobacteria will contribute to the coffee plants once inoculated.
Biocontrol properties of rhizobacteria
-
In this study, Bacillus sanguinis demonstrated HCN production, suggesting its potential role as a biological agent against plant pathogens. HCN is mostly synthesized by Bacillus species, which is believed to disrupt several cellular processes such as the electron transport chain, correct functioning of enzymes and even impedes the action of cytochrome oxidase of the target pathogens[20]. HCN production of phosphate-solubilizing Bacillus isolates was also observed as a biochemical trait of potential biofertilizer agent for coffee production[21].
On the other hand, Bacillus sanguinis and Burkholderia sp. had promising potential as a biocontrol agent due to its capability to produce multiple lytic enzymes, which is an essential strategy for fungal inhibition. Production of lytic enzymes of rhizobacteria is considered as a plant disease inhibitor since these enzymes are involved in cell wall degradation of plant pathogens present in the soil environment[22,23]. Lytic enzymes can be used as biocontrol agents to inhibit fungal pathogens that cause diseases in crops[24].
Stress tolerance of rhizobacteria
-
Abiotic stresses such as salinity, acidity or alkalinity, and heavy metal contamination may have a detrimental effect on agricultural production by affecting plant growth, nutritional imbalance, and physiological and metabolic changes. Rhizobacteria may help in plant growth promotion and alleviation of the stress-induced changes in the host plant[25]. Rhizobacteria that tolerate high concentrations of NaCl can help plants thrive in saline soil through their mechanisms such as osmolytes regulation, nitrogen fixation, solubilization of phosphate, as well as formation of auxin, siderophore and exopolysaccharides[26]. Meanwhile, plants are vulnerable to heavy metal stress exposure, which may came from industrial and other environmental pollutants. Rhizobacteria are essential for the reduction of toxic heavy metals in plants, they can contribute to the improvement of heavy metal tolerance and plant growth through improved phytoremediation and metal accumulation inside the plant[27]. The pH is also considered as a limiting condition for plants but stress tolerant rhizobacteria considerably enhance the seed germination of crops in acidic or alkaline soils[28]. The tolerance of isolates at low pH is expected since the pH of the soil used in the present study is acidic. The ability of the rhizobacteria to withstand a wide range of abiotic stresses indicates their potential to promote plant growth and protect plants from the detrimental effects of abiotic stresses in the soil.
Coffee rhizobacteria as potential biofertilizers
-
The bacterial isolates obtained from the Liberica rhizosphere are Pantoea rwandensis, Bacillus pseudomycoides, and Pantoea sp. that exhibited tolerance to high salt concentration and wide range of pH and heavy metal concentrations, but only B. pseudomycoides exhibited amylase activity. On the other hand, the Robusta rhizobacterial isolates identified in our study were Burkholderia cepacia, Bacillus sanguinis and Burkholderia sp. that showed promising performance, specifically the B. sanguinis that has the ability to produce multiple lytic enzymes and HCN. The result of this study indicates that both Liberica and Robusta rhizosphere contained beneficial rhizobacteria that establish mutual symbiotic relationship with the host plant. Previously, phosphate solubilizing species under the genera Bacillus and Burkholderia and the species of endophytic Pantoea were reported to be abundant in the rhizosphere of forest-grown Coffea arabica[12,29]. These rhizobacterial isolates demonstrated a great potential to be utilized as a biofertilizer to improve the growth and yield of coffee.
The bacterial genus Pantoea was found to be abundant in the rhizosphere microbiome and bean fermentation of coffee[30]. This genus comprises many versatile endophytic species that possess a variety of biosynthetic and biodegradative capabilities. They are found to be useful for biocontrol of plant pathogens, bioremediation of contaminated environments, biosensors, and a source of therapeutic drugs[31]. In addition, Bacillus species was discovered to be a phosphate-solubilizer and enhanced the growth of coffee seedlings and the availability of phosphorus in the soil[32]. Specifically, B. pseudomycoides was found to be a good choice in phytoremediation and an active agent in biofertilizers or biofungicide[33]. Meanwhile, B. sanguinis was similarly found to produce multiple lytic enzymes such as amylase, cellulase, protease, and xylanase[34], indicating its important role against plant pathogens. Lastly, Burkholderia species in general can establish symbiotic relationships with terrestrial plants, functioning as active rhizosphere components, endophytic plant colonizers, or microsymbionts in legume root nodules[35].
-
Our work successfully screened indigenous and beneficial rhizobacteria associated with Robusta and Liberica coffee rhizosphere. They belong to the genera Bacillus, Burkholderia, and Pantoea, which were reported to have plant growth-promoting attributes, biocontrol activities, and tolerance to abiotic stresses. These bacteria can be utilized as an alternative to chemical fertilization and be used as a potential biofertilizer for coffee cultivation in the Philippines. The performance of these isolates as a biofertilizer may vary, but their effectivity can only be verified upon application to crops.
-
All the authors confirm equal contributions for the following: study conception and design, data collection, analysis and interpretation of results, and manuscript preparation.
-
All data generated or analyzed during this study are included in this published article.
The study was funded by the Research Center of Cavite State University, Philippines through the Small Scale CvSU Research Grant. The authors are grateful to CvSU-Research Center for allowing them to conduct experiments in the Bacteriology and Genetics Laboratories of the Interdisciplinary Research Building.
-
The authors declare that they have no conflict of interest.
- Copyright: © 2023 by the author(s). Published by Maximum Academic Press, Fayetteville, GA. This article is an open access article distributed under Creative Commons Attribution License (CC BY 4.0), visit https://creativecommons.org/licenses/by/4.0/.
-
About this article
Cite this article
Navarro GVD, Quirong DD, Maghanoy GA, Cortes AD. 2023. Characterization and identification of rhizobacteria associated with Liberica and Robusta coffee rhizosphere. Technology in Horticulture 3:24 doi: 10.48130/TIH-2023-0024
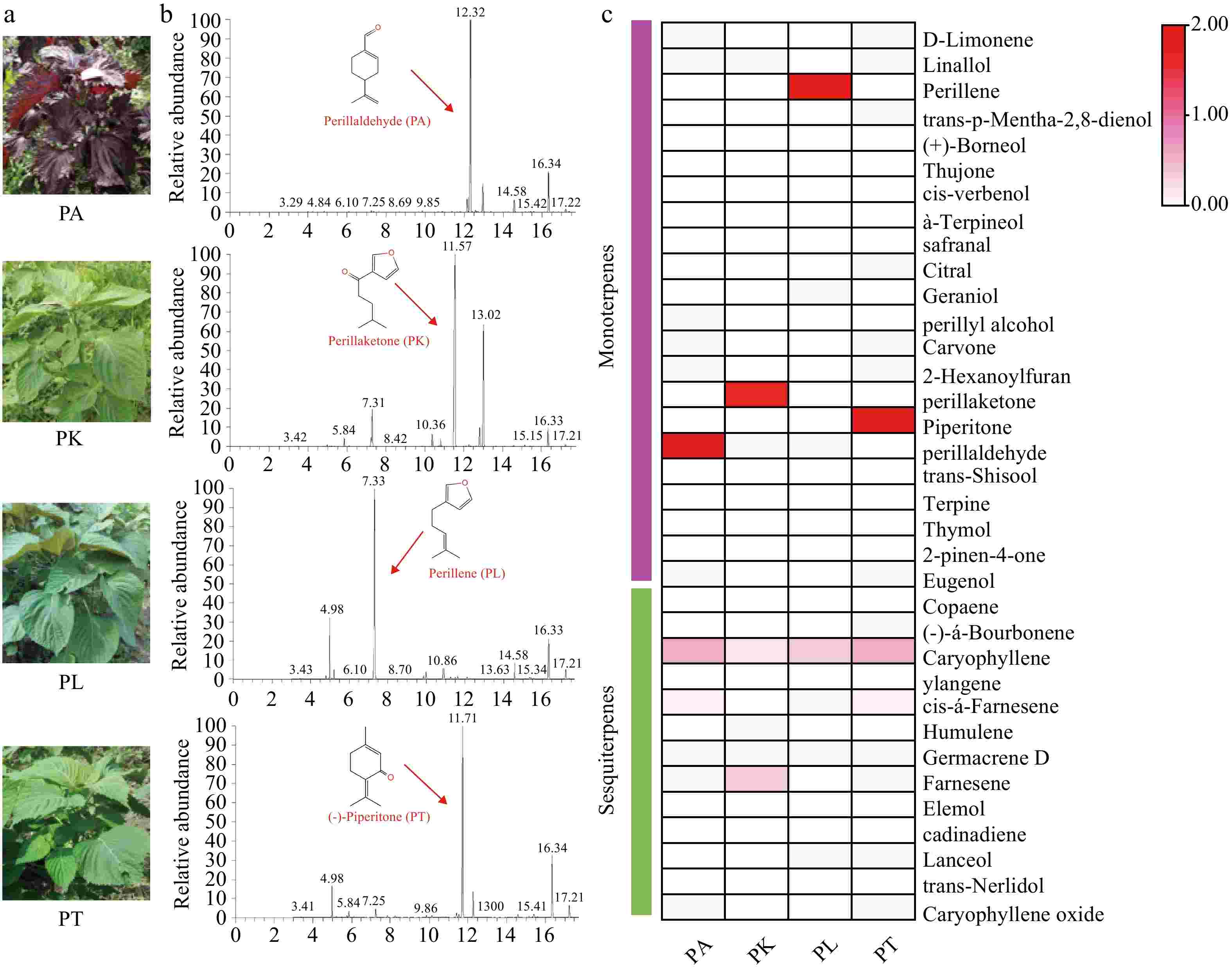