-
The data released by the National Bureau of Statistics indicates that China has exhibited a consistent growth trajectory in fruit production in recent years. This growth has been accompanied by a sustained global leadership position in both the total area of orchards and overall fruit production. By 2023, China's fruit production reached 327.4428 million tons. The fruit industry in China has emerged as the third largest agricultural planting sector, following grains and vegetables, due to the expansion of fruit cultivation areas and a corresponding rise in production levels[1]. China's fruit industry is recognized as a global leader both in terms of production volume and cultivation. However, when compared to developed countries, there exists a significant gap in the technology employed for the deep processing of fruit in China. The relatively short shelf-life of fruits after post-harvest makes them vulnerable to corruption spoilage deterioration, to effectively utilize abundant resources, China has implemented a winemaking strategy that replaces substitutes with fruit[2].
Fruit wines are low-fermented wines produced from fresh fruits through the processes of physical crushing or pressing, followed by either complete or partial fermentation. The alcohol content of these wines typically ranges from 7% to 18% by volume. Fruit wines can be categorized according to their sugar content, which includes dry fruit wines, semi-dry fruit wines, semi-sweet fruit wines, and sweet fruit wines[3]. The fruit wine industry in China is currently undergoing significant growth, characterized by an increasing market size and a heightened level of consumer awareness and acceptance of fruit wines[4]. Fruit wine, acknowledged for its nutritional significance, contains a diverse array of beneficial nutrients that are vital for human health. These components include, but are not restricted to, vitamins, amino acids, trace elements, and minerals. Berry wines not only retain these nutrients during the fermentation process but are also rich in polyphenolic compounds and alcohols, such as anthocyanins, flavonoids, and resveratrol[5]. These substances not only contribute to the unique flavor and color of fruit wines, but they are also highly valued by consumers for their potential health benefits.
The color of fruit wines is a critical determinant influencing consumer purchasing decisions. However, the issue of color instability in fruit wines presents a significant barrier to the growth and development of the fruit wine industry. Consequently, addressing the issue of color stability in fruit wines is essential for fostering sustained growth and facilitating market expansion within the fruit wine sector[6]. Anthocyanin, a compound prevalent in the plant kingdom, serves as the primary pigment responsible for the coloration of fruit wines[7]. Anthocyanin derivatives are synthesized from free anthocyanins, glycolytic intermediates, and certain polyphenolic compounds through a series of reactions, including cyclization, condensation, addition, and polymerization, that occur during the aging process of fruit wines[8]. Depending on their structural characteristics, anthocyanin derivatives can be categorized into four distinct groups: non-acylated anthocyanins, acylated anthocyanins, pyranoanthocyanins, and polymeric anthocyanins[9]. Vinylphenolic pyranoanthocyanins (VPA) are stable derivatives of anthocyanins that are synthesized through the combination of monomeric anthocyanins and hydroxycinnamic acid analogues. These derivatives are distinguished by their low concentration, high stability, and enhanced resistance to SO2 and oxidative degradation[10]. Research has shown that utilizing yeast strains with hydroxycinnamate acid decarboxylase (HCDC) activity in fermentation processes promotes the decarboxylation of hydroxycinnamate, leading to the production of vinylphenols (VPs)[11]. These VPs subsequently interact with grape anthocyanins to produce pyranoanthocyanins, which can substantially enhance the stability of color[10,12]. As the function of HCDC in the development of VPA and its associated mechanisms have become increasingly clarified, research focus has transitioned towards the identification of strains that demonstrate heightened HCDC activity, to improve the color stability of fruit wines[13].
As the understanding of the role of HCDC in the formation of VPA has evolved, researchers have increasingly directed their efforts towards screening strains exhibiting high HCDC activity and optimizing these strains to enhance the quality of fruit wine. This endeavor aims to significantly improve the stability of fruit wine color. In this paper, we review the color protection mechanisms associated with HCDC, the methodologies for evaluating HCDC activity, a comparative analysis of HCDC activity across various yeast strains, and the role of HCDC in the fermentation process. This review serves as a valuable reference for fruit wine production and the identification of high HCDC yeast strains.
-
Phenolic acid decarboxylase (PAD) is a key enzyme in the metabolic pathway of phenolic acid, and it can catalyze the non-oxidative decarboxylation of phenolic acid to generate the corresponding 4-vinyl derivatives[13,14]. PAD can be classified into HCDC and phenylacrylic acid decarboxylase according to the different substrates[14]. Yeasts with HCDC activity can be used to decarboxylate hydroxycinnamic acids (HCAs), and condense with anthocyanins to produce VPA adducts of great colour stability[15,16].
Overview of anthocyanin research
-
Anthocyanins are water-soluble pigment compounds that are prevalent throughout the plant kingdom, particularly in the roots, leaves, flowers, and fruits of various plant species. Anthocyanins serve as the precursor compounds to anthocyanins, which are commonly linked to sugars such as glucose, galactose, and xylose through glycosidic bonds, resulting in the formation of anthocyanins under natural conditions[7,17]. Anthocyanins in the field of food science are primarily categorized into six groups based on the number of hydroxyl substitutions on the B-ring of the anthocyanin structure and variations in methylation. These categories include malvidin, cyanidin, peonidin, pelargonidin, delphinidin, and petunidin. Berries, such as blueberries, mulberries, and grapes, are abundant sources of anthocyanins. These compounds are the principal substances responsible for the coloration of fruit wines and can interact with phenolic compounds, including phenolic acids and flavanols, present in fruit wines[18]. Anthocyanins possess multiple active hydroxyl groups and positively charged ions, contributing to their structural instability. The stability of anthocyanins is also influenced by various environmental factors, including yeast metabolites, pH levels, temperature, light exposure, SO2, as well as the presence of metal ions, glycosidases, and polyphenol oxidase enzymes[6,19].
Structure of anthocyanins
-
The stability of anthocyanins is considerably influenced by their structural characteristics. Typically, an increase in the number of hydroxyl substitutions on the parent nucleus of the anthocyanin 2-phenylbenzopyran cation correlates with a decrease in stability. Conversely, the presence of methoxy substitutions can impede the transition of anthocyanins from the colored form to the colorless methanol pseudo-basic form, thereby enhancing their stability[20]. During the fermentation of fruit wines, metabolites produced by yeast, along with phenolic compounds derived from berries, can interact with anthocyanin monomers to yield anthocyanin derivatives, thereby enhancing their stability. The pyranoanthocyanins generated during the fermentation and aging processes of fruit wines can be classified into five categories, including vitisins-type anthocyanins, methylpyranoanthocyanin, phenylpyranoanthocyanin, flavanolpyranoanthocyanin, and second-generation anthocyanin derivatives, based on the substrates involved in the reactions[21]. Comprehensive details regarding these classifications are presented in Fig. 1[21−24]. The structural characteristics of these derivatives are defined by their foundation on the original anthocyanins, which undergo a cyclization reaction to form an additional pyran ring between the hydroxyl groups located at the C4 and C5 positions[25,26]. The bis-pyranose ring structure offers an increased number of resonance forms for pyranoanthocyanins, thereby enhancing their resistance to oxidative damage, variations in pH, and fluctuations in temperature[12,27,28]. Furthermore, due to the complete saturation of the C4 position in the parent structure of pyranoanthocyanins, these compounds are unable to undergo reactions with HSO3− and exhibit a marked resistance to bleaching effects caused by SO2[23].
Microbial influence on anthocyanin during the brewing process
-
During the fermentation of fruit wine, yeast metabolites engage in interactions with anthocyanins present in grapes, resulting in the formation of derivative pigments. In the winemaking process, the production of acetaldehyde and pyruvic acid facilitates the synthesis of vitisin A and B, which play a significant role in maintaining color stability during prolonged aging. The utilization of yeast strains that exhibit high production levels of pyruvic acid and/or acetaldehyde further promotes the formation of vitisins, thereby contributing to enhanced color stability[29]. Studies have demonstrated that Schizosaccharomyces pombe produces greater quantities of pyruvate during fermentation compared to the commercially utilized Saccharomyces cerevisiae, and a notable production of vitisin A has been observed[30]. The ability of Schizosaccharomyces pombe to produce elevated levels of acetaldehyde has also been documented in cranberry wine[31]. VPA, recognized as a significant anthocyanin derivative in fruit wines, is stabilized by yeast during fermentation by promoting the conversion of monomeric anthocyanins to VPA through the action of HCDC[32,33]. Certain yeast species not only promote the development of more stable anthocyanin derivatives but also contribute to changes in wine color during fermentation by either degrading or producing organic acids[10]. In sequential fermentations utilizing Lachancea percherrima, the total anthocyanin concentration was observed to be higher than that of the Saccharomyces cerevisiae control, with a difference of 8%−10%. This increase in anthocyanin color intensity can be attributed to the acidification of the wine caused by lactic acid produced by L. percherrima during fermentation[34,35].
Currently, numerous studies have been conducted to enhance the color of fruit wines. Among these investigations, the cochromatic effects of phenolic compounds on anthocyanins, as well as the comparative analysis of the effects of various cochromes, have received the most attention[36,37]. In recent years, the application of yeast metabolites, specifically yeast-produced HCDC, to enhance the formation of structurally more stable anthocyanin derivatives during fermentation has emerged as a prominent area of research.
Sources of HCDC
-
HCAs are classified as secondary metabolites present in both plant and fungal species, characterized by their C6-C3 structural arrangement[38]. Ferulic acid, p-coumaric acid, and caffeic acid are commonly occurring HCAs that undergo decarboxylation, resulting in the formation of VPs, specifically 4-vinylphenol, and 4-vinylguaiacol[39]. Subsequently, these VPs are reduced to form ethylphenols (EPs), namely 4-ethylphenol and 4-ethylguaiacol[40].
Numerous species of microorganisms have been documented to decarboxylate HCAs. Specifically, the decarboxylation of ferulic acid, p-coumaric acid, and caffeic acid by Saccharomyces cerevisiae, along with various bacterial and fungal species, has been substantiated[41]. Cavin et al.[42] have successfully purified p-coumaric acid decarboxylase from Lactobacillus plantarum, demonstrating that the enzyme also exhibits activity on caffeic acid. The decarboxylase has an apparent molecular mass of 93 kDa and is characterized as a tetramer composed of four subunits, each with a mass of 23.5 kDa[41]. Some researchers identified Pseudomonas fluorescens as a source for the purification of ferulic acid decarboxylase, which subsequently resulted in the isolation of Brucella pumilus[43]. The purified decarboxylase was found to exist as homodimers with an apparent molecular mass ranging from 45 to 40.4 kDa[43]. Huang et al.[44] were the first to clone, sequence, and express the phenolic acid decarboxylase gene in Candida guilliermondii. This organism was shown to convert the primary substrates, ferulic acid, and p-coumaric acid, into their respective products. Furthermore, ultrafiltration of the cell-free extracts significantly enhanced the decarboxylation of ferulic acid by the purified enzyme, whereas it had negligible effects on the decarboxylation of coumaric acid. Lactic acid bacteria (LAB) are also characterized by the presence of HCDC, which is essential for the decarboxylation process, especially in the production of cheese and yogurt. The HCDC enzyme in LAB catalyzes the conversion of HCA to 4-vinylphenol, thereby influencing the organoleptic properties of the final product[38]. LAB are known to produce HCDC during the fermentation of wine. This process involves the decarboxylation of p-coumaric acid and ferulic acid, resulting in the formation of 4-vinylphenol and 4-vinylguaiacol. HCDC mRNA expression has also been shown to be induced by the addition of p-coumaric acid or ferulic acid to the cultures[45].
In the production of fruit wine, both Saccharomyces cerevisiae and non-Saccharomyces cerevisiae are capable of secreting HCDC. However, their activity is dependent on the specific strain. Generally, non-Saccharomyces cerevisiae exhibits greater enzymatic activity compared to Saccharomyces cerevisiae and play a significant role in the fermentation process. Edlin et al.[41] successfully isolated HCDC from Brettanomyces anomalus, an enzyme that facilitates the decarboxylation of p-coumaric acid, caffeic acid, and ferulic acid, resulting in the production of 4-hydroxystyrene, 3,4-dihydroxystyrene, and 3-methoxy-4-hydroxystyrene. These resultant compounds are then further reduced to form their respective ethyl derivatives. Godoy et al.[46] purified p-coumarate decarboxylase from Brettanomyces bruxellensis, revealing a molecular weight of 21 kDa under denaturing conditions, in contrast to a mass of 41 kDa under native conditions. The enzyme exhibited optimal activity at a temperature of 40 °C and a pH of 6.0. Richard et al.[47] discovered that the activity of HCDC can be significantly enhanced in Saccharomyces cerevisiae through the overexpression of PAD1 and FDC1. Subsequent research has increasingly focused on HCDC in yeast, revealing its widespread presence in Saccharomyces cerevisiae, while also demonstrating strain-dependent variations in non-Saccharomyces cerevisiae. In addition to the widely researched species B. anomalus, HCDC has also been identified in Metschnikowia pulcherrima, Wickerhamomyces anomalosus, Torulaspora delbrueckii, Pichia guilliermondii, and Schizosaccharomyces pombe[10,48−50]. The subjects exhibited elevated enzyme activity, which is crucial for the fermentation process of fruit wines.
Mechanism of action of HCDC in VPA synthesis
-
Anthocyanins serve as the primary pigments responsible for coloration in fruit wines, with the specific types of anthocyanins differing among various fruit sources. In grapes, the predominant anthocyanins are malvidin-3-O-glucoside and delphinidin-3,5-O-diglucoside[51]. In mulberries, the main anthocyanins include cyanidin-3-O-glucoside and cyanidin-3-O-rutinoside[52]. Blueberries primarily contain cyanidin and delphinidin[53]. The coloration of fruit wines is influenced by the content, type, and form of anthocyanins present. Based on their state of existence, anthocyanins in fruit wines can be categorized into free anthocyanins, co-pigmented anthocyanins, and polymeric anthocyanins. It is generally acknowledged that the coloration of fresh fruit wines is predominantly influenced by free anthocyanins, while polymeric anthocyanins significantly contribute to the color characteristics of fruit wines as they undergo aging. The formation of stabilized polymeric anthocyanins effectively mitigates color degradation in fruit wines over extended aging periods[2].
Derivatization refers to the process through which anthocyanins are transformed into polymeric anthocyanins and pyranoanthocyanins. Typically, polymeric anthocyanins exhibit a purplish-red hue, whereas pyranoanthocyanins are more likely to display a yellow coloration[54]. Pyranoanthocyanins are important derivatives of anthocyanins that play a crucial role in the coloration of fruit wines. VPA is a stabilized pyranoanthocyanin found in aged Port wines, characterized by its reddish-purple coloration. Research indicates that VPA is synthesized through a multi-step condensation process involving HCA compounds derived from grapes during the fermentation and aging stages[55].
As the study advanced, it was observed that certain strains exhibited HCDC activity during fermentation, potentially facilitating the formation of VPA. The underlying mechanism is as follows: HCA undergoes decarboxylation by HCDC, resulting in the production of the highly reactive compound 4-vinylphenol. This compound subsequently undergoes rapid condensation with anthocyanin to yield VPA[12]. Simultaneously, specific strains of yeast exhibit vinylphenol reductase (VPR) activity, which aids in the conversion of the intermediate compound vinylphenol into 4-ethylphenol. This enzyme has been shown to negatively affect the quality of fruit wines[10]. The mechanism of action of HCDC is shown in Fig. 2. The intricate structure of VPA, characterized by five aromatic rings, allows for its effective separation from fruit wine through UHPLC, where it is detected at the end of the chromatogram[56]. Taking VPA in wine as an example, the principal parameters of UV-visible absorption and mass spectrometry of the most common VPA are presented in Table 1.
Table 1. The main VPAs formed by yeast fermentation in wine.
-
The activity of the enzyme is dependent on the strain and can vary significantly among different strains. Compared to Saccharomyces cerevisiae, certain non-Saccharomyces cerevisiae strains, such as S. pombe and P. guilliermondii, are more effective in promoting the formation of VPA[30,48].
Common methods for HCDC activity assessment
-
Currently, the available research on assays for HCDC activity is relatively limited. Visible color change is usually the preferred strategy for rapid detection and screening, and the most frequently employed technique for evaluating enzyme activity is based on the chromogenic properties of solid media[50,58]. This method is predicated on the decarboxylation reaction of HCA, which induces an increase in the pH of the medium, thereby alkalinizing the environment. Consequently, this alteration results in a color change of the medium from yellow to purple. This colorimetric shift can serve as a preliminary indicator of the activity strength of the strain HCDC[16,59]. However, HCDC is an intracellular enzyme, and the preparation of its enzyme extract presents significant technical challenges, thereby limiting the widespread applicability of this method. Zhou et al.[50] streamlined the procedures established in prior research by directly inoculating a single colony for preliminary identification, thereby significantly reducing the operational complexity. Deng et al.[60] simplified the experimental procedure by utilizing a liquid medium and collecting samples at various time intervals to monitor changes in color and pH.
In addition, HPLC has been extensively employed in activity detection owing to its high accuracy, rapid analytical capabilities, and user-friendly operation[50]. Most studies employed a 96-well plate fermentation system in conjunction with HPLC analysis. This approach not only more accurately reflects real fermentation conditions but also ensures consistency in the inoculum volume, thereby enhancing the accuracy of comparisons among different strains[61]. This method reduces the workload associated with sieving and enhances overall efficiency. Previous studies have employed a combination of assays to evaluate enzyme activity, initiating with solid medium colorimetry for the preliminary assessment, followed by HPLC for the specific enzyme activity analysis. This approach not only streamlines the operational process but also facilitates the comparison of enzyme activity across various strains[50].
In addition, a subset of researchers have used UV spectrophotometry for enzyme activity assays[42], which facilitates precise measurement of changes in substrate absorption and provides a high-throughput method for assessing varying acid decarboxylation capabilities, thereby eliminating the need for additional preparation or extraction[38]. Although this method is currently underutilized in yeast, its successful application in detecting decarboxylase activity in Lactobacillus suggests significant potential for further development. Table 2 provides a summary of the methods that are currently most employed for assessing HCDC activity.
Table 2. Methods of HCDC activity assessment.
Method Characteristic Experimental scheme Ref. Solid medium colorimetric method (1) Detection and analysis of results are quick and easy;
(2) Pre-treatment for the preparation of enzyme extracts is more complicated;
(3) Color variations may be influenced by a range of factors, such as temperature, pH levels, and the specific composition of the medium.LB agar plates were prepared containing 0.01% (w/v) bromocresol violet and supplemented with 0.145% (w/v) of ferulic, p-coumaric, or caffeic acids, with the pH adjusted to 5.25 using 5% isopropanol. Subsequently, 10 μL aliquots of cellular extracts, which were prepared in a 50 mM phosphate buffer (pH = 6.0), were incubated for 1−2 h at 37−45 °C, and the color change from yellow to purple indicated the presence of HCDC activity. [59] Cellular extracts cultured for 24 h in a 10 mM phosphate buffer (pH = 7.0) were aliquoted (10 μL) and subsequently spread onto YPD plates containing 0.01% (w/v) bromocresol violet, 0.145% (w/v) ferulic acid, or p-coumaric acid. The plates were then incubated at 37 °C for a duration of 1−2 h. [16,62] Yeast was inoculated at the center of YPD plates that contained 0.01% (w/v) bromocresol violet and were supplemented with 0.145% (w/v) p-coumaric acid. The plates were subsequently incubated for 24 h at 37 °C. [50] A 5 mM solution of ferulic acid, supplemented with 0.1% (w/v) bromocresol violet, was utilized for the experiment. Yeast pre-cultures were introduced to the solution, and samples were collected at intervals of 0, 5, 10, 20, 40, and 60 min. The enzymatic activity was assessed by observing the color change from yellow (pH = 5.2) to purple (pH = 6.8). [60] HPLC analytical method (1) Accurately determining and comparing enzyme activity sizes;
(2) Suitable for large-scale sieving of bacteria;
(3) Better control of the consistency of bacterial inoculum;
(4) Saving reagents;
(5) Higher costs.1 mL of yeast (108 cfu/mL) was inoculated in 10 mL of YEPD medium containing 115 mg/L p-coumaric acid in test tubes. Following a fermentation period of 10 d, the supernatant was filtered through a 0.45 μm filter and then analyzed by HPLC. [15,48] Yeast (108 cfu/mL) was inoculated with 1 mL of yeast (108 cfu/mL) in 10 mL of YEPD medium supplemented with 50 mg/L p-coumaric acid. Following a fermentation period of 10 d at 25 °C, the supernatant was filtered through a 0.45 μm filter and then analyzed by HPLC. [63] A sterile medium was prepared consisting of 0.67% (w/v) commercial yeast nitrogen base (YNB), 2% (w/v) glucose, and 100 mg/L p-coumaric acid. A total of 1.35 mL of this medium was dispensed into deep-well microtiter plates and inoculated with 150 μL of yeast pre-culture (OD600 = 1). After a 10-d incubation period, the samples were centrifuged at 5000 rpm for 10 min to obtain the supernatant, which was analyzed by HPLC. [10,64] YPD liquid screening medium utilized in this study comprised 1% yeast extract, 2% peptone, and 2% glucose, supplemented with 0.145% p-coumaric acid, ferulic acid, and caffeic acid, respectively. Yeast precultures were inoculated into the screening medium at a concentration of 1% and incubated at 30 °C with shaking at 200 rpm for 72 h. Following incubation, the cultures were centrifuged at 5,000 rpm and 4 °C for 5 min. The resulting supernatant was filtered through a 0.22 μm filter and then analyzed by HPLC. [65] The YPD liquid medium was prepared with 0.01% (w/v) bromocresol violet and supplemented with 0.145% (w/v) of caffeic acid, p-coumaric acid, and ferulic acid. Subsequently, 0.1% (v/v) of yeast was inoculated into the YPD medium and incubated for 72 h at 30 °C, 200 rpm before centrifugation at 5,000 g for 5 min and the supernatant was filtered through a 0.45 μm filter, followed by HPLC for analysis. [50] Ultraviolet–visible spectrophotometry (1) More complex to handle, but require time-consuming sample processing or purification;
(2) Only the amount of phenolic acid consumption can be measured, not the amount of vinylphenol produced;
(3) It is used more in lactic acid bacteria to determine enzyme activity and less in yeast.The assay mixture consisted of 25 mM phosphate buffer (whole cell pH = 5.0, protein extract
pH = 6.0) and 1.2 mM substrate (different phenolic acids). The samples were incubated at 30 °C, diluted 20-fold with 25 mM Tris buffer containing 0.3% SDS to block activity and absorbance was measured using the supernatant.[42] Bacteria were grown in MRS broth for 18−24 h at OD = 1.0. Cells were harvested by centrifugation and resuspended in 70 mM sodium phosphate buffer (pH = 6.0) containing 100 μg/mL of ferulic acid or p-coumaric acid to an OD = 1.0. The cells were incubated for 8 h at 30 °C, with centrifugation performed hourly. The supernatant was maintained on ice until further analysis. The concentration of p-coumaric acid was derived by measuring the absorbance at 286 nm, while the concentration of ferulic acid was derived by measuring absorbance at 284 and 312 nm. [45] Determination of the enzymatic activity of cell-free extracts: The standard condition was an assay mixture containing 1.2 mM p-coumaric acid and 100 μL of cell extract (final volume of 1.5 mL sodium phosphate buffer, pH = 6.0). After adding the cell-free extract and incubating at 37 °C for 26 h, 20 μL of the sample was diluted 20-fold in 380 μL of termination buffer (25 mM Tris buffer containing 0.3% sodium dodecyl sulfate) to terminate the enzyme activity, and the concentration of p-coumaric acid was derived by measuring the absorbance at 286 nm. [66] Determination of the enzymatic activity of resting cell suspensions: standard conditions were assay mixtures containing 1.2 mM p-coumaric acid and 109 cfu/mL (final volume of 1.5 mL McIlvaine buffer, ph = 4.0). After adding washed cells and incubating for 22 h at 30 °C, 40 μL of the sample was diluted 20-fold in 760 μL of termination buffer and centrifuged at 12,000 g for
2 min, and the absorbance was determined using the supernatant.CDM medium was used: 0.1% K2HPO4, 0.1% KHPO4, 0.5% yeast extract, 0.025% MgSO4, 0.0005% NaCl, 500 mg/L p-coumaric acid, and 0.5% glucose. Bacteria were incubated in MRS broth overnight (32 °C), centrifuged at 8000 g for 10 min at 4 °C, cultures were collected and washed twice with sterile saline (0.085% NaCl, pH = 7.0) and reconstituted, and inoculated with CDM in 96-well plates and incubated at 32 °C for 36 h. The 96-well plates were centrifuged at 4,000 g for 30 min at room temperature and the supernatants were collected immediately and frozen. The supernatant was immediately collected and frozen, and the absorbance was measured after dilution with buffer solution. [38] Factors affecting HCDC enzyme activity
-
The activity of HCDC, similar to that of other enzyme types, is influenced by various factors, including temperature, pH, nutrient availability, and ethanol concentration. Each of these variables plays a crucial role in determining the enzymatic activity of HCDC[67].
The fermentation of fruit wines is generally performed at temperatures below 30 °C. However, it is important to recognize that different sources of HCDC exhibit varying optimal temperatures and temperature tolerances. The optimal temperature for HCDC purified from B. anomalus was determined to be 40 °C. Notably, its enzymatic activity rapidly declined when the temperature exceeded 40 °C; however, approximately 12.5% of the activity observed at 40 °C remained detectable at 60 °C[41]. The enzymatic activity was significantly diminished in the extracts stored at 4 or 20 °C, with only 49.3% of the initial activity preserved after 4 d at 20 °C and 46.8% retained after 7 d at 4 °C. Research has shown that enzyme stability is sustained for an extended duration when extracts are frozen at −20 °C[41]. The optimal temperature for p-coumarate decarboxylase in D. bruxellensis was determined to be 16 °C. In contrast, the optimal temperature for HCDC in D. bruxellensis D37 was found to be 20−30 °C, with diminished enzyme activity noted at 15−20 °C. No enzymatic activity was detected at 30−40 and 0−15 °C[67]. The observed interspecific and intraspecific variation is associated with the strain specificity of the yeast.
Changes in pH can significantly influence enzyme activity, with each enzyme exhibiting an optimal pH at which its activity is maximized. Deviations from this optimal pH result in a reduction of enzyme activity. The purified HCDC was evaluated for pH tolerance, revealing that the purified decarboxylase demonstrated an optimal pH of 6.0 and maintained activity within the pH range of 4−8[41]. Valdetara et al.[70] demonstrated that the optimal pH for the expression of the cinnamic acid decarboxylase gene is 4.5. At an SO2 concentration of 0.25 mg/L and an ethanol concentration of 8.75% (v/v), maximum gene expression occurs. Under these conditions, the strain exhibited enhanced production of HCDC and demonstrated increased activity and efficiency in transformation. D. bruxellensis effectively converted p-coumaric acid to 4-ethylphenol at an optimal pH of 2.5. In the absence of other limiting factors, D37 exhibited no HCDC activity within the pH range of 1.75−2.17[67]. Juice typically contains a higher concentration of alpha hydroxy acids, with a pH range between 2.0 and 4.0[68]. The strain that produces HCDC exhibits enhanced acid tolerance, demonstrating increased activity under these conditions. Furthermore, the HCDC gene is effectively expressed, leading to the production of a greater quantity of enzymes, which enhances the efficiency of fermentation processes.
A significant quantity of nutrients, primarily fermentable sugars such as glucose, fructose, and sucrose, are present in the fermentation system, and these nutrients influence enzyme activity. Benito et al.[67] conducted a study examining the ability of D. bruxellensis to transform p-coumaric acid into 4-ethylphenol under different concentrations of glucose. The highest HCDC activity was recorded at a glucose concentration of 150 mg/L. The research conducted by Dias et al.[69] demonstrated that the conversion rate was 92.5% in a medium supplemented with 20 g/L of glucose, while it was only 6.3% in a medium supplemented with 20 g/L of alginate. Adding fructose also appeared to have little effect on converting.
Ethanol is a byproduct of alcoholic fermentation conducted by yeast. The accumulation of ethanol during the fermentation process inhibits enzyme activity, and the inhibitory effect of ethanol on HCDC activity intensifies with increasing concentrations. D. bruxellensis demonstrated an efficiency of approximately 90% in the presence of 14.5% (v/v) ethanol. In contrast, D37 exhibited no HCDC activity when the ethanol concentration exceeded 15% (v/v). Furthermore, a concentration of 10% (v/v) ethanol resulted in a delay of more than three days in the formation of 4-ethylphenol when compared to a concentration of 5% (v/v)[67]. In the context of the expression of putative decarboxylase genes, an elevation in ethanol concentration negatively impacts the availability of transcripts or their average stability[70]. Godoy et al.[46] demonstrated that the activity of HCDC was significantly diminished following a brief incubation period in the presence of 10% (v/v) and 12% (v/v) ethanol. Specifically, the enzyme exhibited a 50% reduction in activity after 10 min of incubation, ultimately resulting in a complete loss of activity after 1 h. In contrast, fruit wines generally possess a lower alcohol content, typically 2%−5% (v/v), whereas traditional wines typically exceed 12% (v/v)[71]. HCDC is more effective in stabilizing the color of fruit wines with low alcohol concentrations in comparison to those with high ethanol concentrations.
In addition to the aforementioned factors, metal ions, and certain additives also induce alterations in enzyme activity. Edlin et al.[41] showed that Cr3+, EDTA, and Mg2+ significantly enhanced the activity of purified HCDC. In contrast, Zn2+, Ca2+, Co2+, Mn2+, Li+, and Cu2+ exhibited partial inhibition of the enzyme activity, whereas Fe3+, Ag+, and SDS resulted in complete inhibition of decarboxylase activity. Several studies have shown that the presence of p-coumaric acid, ferulic acid, and cinnamyl esterase (CE) in the culture medium results in an enhancement of HCDC activity[46,63,69,70]. Inhibitors exhibited a significant inhibitory effect on enzyme activity. Notably, D. anomala demonstrated a complete absence of decarboxylase activity when the concentration of sorbic acid exceeded 750 mg/L or when the concentration of nicotinic acid surpassed 25 mg/L[67]. SO2 influenced enzyme activity and impacted cell viability, B. bruxellensis exhibited no viable cells after 8 h of incubation and demonstrated a complete absence of HCDC enzyme activity at a concentration of 100 mg/L total SO2. After 6 h of incubation, the enzyme activity was reduced to one-third of its initial level. At sulfite concentrations of approximately 75 mg/L total SO2, the yeast exhibited a partial loss of activity. In contrast, at higher concentrations (at least 75 mg/L total SO2), the majority of the yeast populations experienced a decline in viability; however, they retained a portion of their enzyme activity[66]. In addition, the overexpression of PAD1 and FDC1 in Saccharomyces cerevisiae resulted in a significant increase in cinnamic acid decarboxylase activity. Strains exhibiting the overexpression of these genes displayed tolerance to cinnamic acid concentrations reaching 10 mM; however, this increased tolerance was not evident when FDC1 was overexpressed independently[47].
Numerous factors influence the activity of the HCDC enzyme, but current research on the conditions affecting HCDC enzyme activity in yeast is relatively limited and predominantly concentrated on gene expression and Brettanomyces/Dekkera. Therefore, when screening yeast strains, it is essential to investigate the fermentation characteristics inherent to each strain, as well as to evaluate the adaptability of the HCDC produced by the strain to the fermentation environment.
Comparison of HCDC enzyme activities of different yeasts
-
Most strains of Saccharomyces cerevisiae, as well as non-Saccharomyces cerevisiae, possess the ability to synthesize and release HCDC. However, non-Saccharomyces cerevisiae exhibits significant strain-specific variability, leading to both interspecific and intraspecific differences in the enzyme activity of HCDC produced by these strains. The yeasts that have been reported to exhibit HCDC activity to date include Saccharomyces cerevisiae, Metschnikowia pulcherrmia, Wickerhamomyces anomalus, Lachancea thermotolerans, Torulaspora delbrueckii, Pichia guilliermondii, Zygosaccharomyces bailii, Debaryomyces Hansenii, Issatchenkia terricola, and Candida[10,41,46,48−50,64], the activity level of the HCDC enzyme exhibits variability (Table 3).
Table 3. Comparison of HCDC activities in different yeast.
Yeast species Strains code HCDC activity (%) Ref. S. cerevisiae CL1 49.66 ± 0.91 [61] CL7 17.81 ± 2.29 CL34 14.49 ± 1.11 I34 69.20 ± 2.07 I42 69.99 ± 1.97 I43 78.49 ± 0.14 I56 66.41 ± 11.62 B42 63.35 ± 0.50 B43 63.70 ± 0.56 C9 13.46 ± 1.17 H50 44.32 ± 1.62 H44 4.80 ± 1.29 H63 10.15 ± 1.65 H66 4.58 ± 2.83 F30 75.81 ± 0.50 LH21 9.49 ± 1.08 D35 18.17 ± 1.14 D37 11.95 ± 0.25 D41 14.48 ± 0.70 E35 12.80 ± 0.35 E37 11.74 ± 0.68 FS10 13.58 ± 0.48 FS14 13.09 ± 0.36 YTH41 33.23 ± 0.02 A1 4.29 ± 1.49 A2 14.53 ± 0.10 A4 15.19 ± 1.17 7VA 69.9 [63] 4CV 62.3 TP2A16 81.3 VR5 73.3 AWRI 1503 99.8 71B 65.8 EC1118 97.2 M29 67.9 ZIM2180 66 [64] FPC 5.1 I. terricola FS27 2.62 ± 1.92 [61] FS5 4.96 ± 3.01 H5 5.80 ± 1.21 W. anomalus D6 78.71 ± 0.05 S138 91.1 [64] Y5 14.32 [50] M. pulcherrima B18 18.19 ± 2.11 [61] B19 18.20 ± 5.44 B20 15.67 ± 2.83 B40 34.88 ± 0.01 E28 8.88 ± 0.58 C14 14.32 ± 0.05 Y1 3.52 [50] Y2 3.35 Y3 2.56 Y4 6.46 MC1 − [16] MC4 − MC6 − M. reukaufii Sut85 86 [64] Z. bailii I20 6.64 ± 0.51 [61] I29 7.98 ± 0.57 D. hansenii Sut116RT 41.3 [64] P. manshurica M49 66.9 P. guilliermondii ZIM624 88.6 C. fabianii Y6 3.13 [50] Y7 2.75 Y8 4.07 Y9 2.85 T. delbrueckii Y10 8.41 The demonstration of HCDC activity (%) through the utilization of p-coumaric acid indicates that a value of "−" signifies the absence of a specific activity value reported in the literature. Overall, several yeast strains demonstrated significant HCDC activity. Notably, both Saccharomyces cerevisiae (including strains I43, F30, 7VA, AWRI1503, and EC1118) and non-Saccharomyces cerevisiae (such as W. anomalus D6, S138, Y5, M. pulcherrima B40, P. manshurica M49, P. guilliermondii ZIM624, and D. hansenii Sut116RT) serve as promising sources of HCDC for fermentation processes. However, the study exclusively measured the HCDC activity of the yeasts, while other enzymatic activities were not assessed. Consequently, it remains unclear whether these yeast strains possess additional capabilities that could enhance the quality of fruit wines. Presently, most of the research has concentrated on the direct effects of mixed fermentation on the quality of fruit wines, with comparatively less emphasis on the HCDC activity of the yeasts. Gaining insights into the fermentation outcomes is undoubtedly crucial for the industry from a practical application standpoint, as is comprehending the underlying mechanisms involved. Monitoring the enzyme production of specific yeast strains through distinct fermentation tests represents the most effective method for evaluating their individual enzyme production capacities[72].
-
Recent studies examining the influence of HCDC on the quality of fruit wines can be divided into two main domains. Firstly, HCDC is found to enhance the color stability of fruit wines through the promotion of VPA formation. Secondly, it positively affects the aroma profile of fruit wines by reducing the levels of ethylphenolic compounds, which are recognized for imparting unfavorable flavors to the wine.
Effect on color stability
-
The role of HCDC in the formation of VPA, a stable pigment that exhibits greater resilience to variations in pH, oxidation, and SO2 bleaching compared to anthocyanins under winemaking conditions, has been elucidated in previous chapters. Consequently, HCDC produced by yeast contributes positively to the color stability of fruit wines[25,33]. The predominant form of HCAs present in grapes is as tartaric acid esters (TE-HCAs). Tartaric, coumaric, and ellagic acids serve as reservoirs for HCAs, which can be liberated through hydrolysis during the aging process. The application of cinnamate lyase during fermentation facilitates the release of HCAs, while yeast exhibiting HCDC activity transforms HCAs into VPs. These VPs are subsequently converted into VPAs through a condensation reaction with anthocyanins[63]. Bozic et al.[64] conducted a screening for high HCDC activity during wine fermentation to isolate P. guilliermondii ZIM624 and W. anomalus S138. The concentrations of VPA synthesized were measured at 40.2 and 38.5 mg/L in monoculture fermentation, 16.4 mg/L in co-culture, and 29.8 mg/L in sequential fermentation, respectively. Their study also showed that the concentration of malvidin-3-O-glucoside-4-vinylphenol derived from the fermentation of ZIM624 was 50 times greater than that obtained from wine artificially aged at 30 °C[49]. It has been shown that the sequential fermentation of P. guillermondii 513 with Saccharomyces cerevisiae results in the production of highly stable vinylphenolic acid anthocyanins, which enhance the stability of wine color[48]. Starmerella bacillaris has been shown to enhance the color of wine. The sequential fermentation of S. bacillaris FA18 with Saccharomyces cerevisiae SCE16 mitigated the rapid transition to orange-brown that is frequently observed in single-varietal wines, such as Primitivo, Negramaro, and Aleatico[73].
Research has predominantly focused on highly expressed HCDC yeasts in the context of wine production, but yeasts exhibiting HCDC activity can also be utilized in the production of other fruit wines to enhance their color. Zhou et al.[50] utilized W. anomalus Y5, which exhibits high HCDC activity, for the fermentation of blueberry wine. This process resulted in the formation of four additional VPAs in the blueberry wine compared to blueberry juice, thereby contributing to a more stable coloration of the wine. The four identified VPAs include cyanidin-3-O-galactoside/glucoside-4-vinylcatechol, cyanidin-3-O-galactoside/glucoside-4-vinylsyringol, malvidin-4-vinylcatechol, and malvidin-4-vinylguaiacol[50]. Based on this foundation, the researchers successfully demonstrated the expression of the gene PAD in Saccharomyces cerevisiae utilizing enzyme surface display technology. This approach resulted in an increase in three anthocyanidins present in blueberry wine: petunidin-3-O-glucoside-4-vinylguaiacol, petunidin-3-O-glucoside-4-vinylphenol, and malvidin-3-O-glucoside-4-vinylguaiacol[60]. Gao et al.[10] conducted a screening of wild yeasts exhibiting high HCDC activity, resulting in the identification of a strain of Saccharomyces cerevisiae I43 and a strain of W. anomalus D6. The sequential fermentation using these two strains demonstrated an enhancement in the production of stabilizing pigments, specifically cyanidin-3-O-glucoside-4-vinylphenol and cyanidin-3-O-rutinoside-4-vinylphenol. These pigments were found to have a stabilizing effect on the color of mulberry wine throughout the fermentation and vinification processes.
Overall, HCA decarboxylation and subsequent condensation with monomeric anthocyanins facilitated the production of more stable VPA by HCDC. This procedure improved the color stability of fruit wines throughout fermentation and subsequent aging, successfully addressing the color variations linked to the instability of anthocyanins. This advancement not only presents an innovative approach for the fruit wine sector to bolster the color stability of their offerings but also lays a scientific groundwork for a more comprehensive understanding of the influence of yeast strains on the formation of pigments in fruit wines. Future investigations may further examine the application of HCDC-active yeasts across various fruit wines and explore methods to optimize the performance of these yeasts through genetic engineering and other techniques to achieve more efficient and stable color enhancement.
Effects on aroma
-
The emergence of off-flavors during the winemaking process constitutes a multifaceted issue that encompasses several stages and a variety of microorganisms. Off-flavors that compromise the quality of wine can be generated before fermentation, throughout the fermentation process, during storage, and after bottling. The primary off-flavors identified include ethyl acetate, H2S, acetaldehyde, and volatile phenols. Yeast plays a significant role in mitigating off-flavors through various mechanisms, including biocompetition, the production of acidity, competition for nutrients, depletion of off-flavor precursors, and adsorption onto the cell wall[63,74−78].
The production of EPs, particularly 4-ethylphenol and 4-ethylguaiacol, has a detrimental effect on the quality of wines. These compounds are frequently characterized by flavors as 'leathery', 'smoky', and 'stable' flavors[79]. EPs are closely associated with the levels of precursors present in the wine, especially HCAs or TE-HCAs[79]. These compounds undergo decarboxylation, catalyzed by HCDC, resulting in the formation of VPs. Subsequently, these VPs are reduced by the VPR to produce EPs[75]. The concentration of EPs generated during fermentation is closely associated with the levels of precursors present in the wine. Additionally, the quantity of EPs produced during fermentation exhibits variability. Cabrita et al.[40] conducted a study examining the transformation of p-coumaric, ferulic, and caffeic acids into 4-ethylphenol, 4-ethylguaiacol, and 4-ethylcatechol throughout the fermentation process. Among the three acids examined, caffeic acid was utilized the least by the yeasts, resulting in the lowest production of 4-ethylcatechol. To regulate the production of EPs, researchers have proposed a range of techniques, including non-thermal methods, the use of additives, and biotechnological approaches[80]. Among the various yeast species, many strains of Saccharomyces cerevisiae and non-Saccharomyces cerevisiae exhibit high levels of HCDC activity while lacking VPR activity. As a result, the employment of yeasts exhibiting high levels of HCDC activity represents a valuable and natural biological resource. These yeasts possess the ability to transform a significant proportion of HCAs into VPs while preventing the subsequent conversion of VPs into EPs, thus reducing the occurrence of off-flavor compounds in wine.
Morata et al.[63,75] used strain expressing esterase activity, which demonstrated an increased concentration through the application of CE treatment. The synergistic effect of these methods resulted in a significant reduction in the combined levels of EPs in the wines. This finding suggests that an enzymatic-biochemical approach, when employed in conjunction with HCDC+ yeast strains, may serve as an effective strategy to safeguard wines from contamination by Brettanomyces/Dekkera, which is known to produce EPs. In a study examining the fermentation of red grape musts using ten commercial yeasts with confirmed high HCDC activity, as well as D. bruxellensis, the levels of 4-ethylphenol were found to 22−498 μg/L. These concentrations were either below or near the sensory threshold for 4EP in wine. In contrast, the control yeasts, which exhibited no HCDC activity, produced 4EP levels of 1,150 μg/L, exceeding the sensory threshold by more than a factor of two[63]. Furthermore, the application of adsorbents, such as activated carbon, may diminish the concentration of EPs but could also influence the volatile compounds and coloration of the wine. Conversely, the reduction of EPs was more pronounced during fermentation with HCDC+ yeast, with the efficacy of removal exhibiting a positive correlation with HCDC activity. The synthesis of malvidin-3-vinylguaiacol contributes to the inhibition of 4-ethylguaiacol formation, thereby enhancing both the color and quality of the wine while simultaneously eliminating undesirable off-flavors[15].
The highly expressed HCDC yeast has demonstrated its efficacy as a biological agent in mitigating the production of EPs. In practical applications, various strategies for controlling EPs production must be considered, including the selection of appropriate yeast strains, the optimization of fermentation conditions, and the application of enzyme treatments. In addition, future research may provide valuable insights into improving the overall quality of wines by refining the fermentation process.
-
The highly expressed HCDC yeast presents a promising approach to the challenges encountered in modern winemaking. It effectively mitigates the problem of unstable coloration in fruit wines while concurrently inhibiting the development of off-flavors that may detract from the overall quality of the wines. HCDC-expressing yeast possesses the capability to decarboxylate HCA into vinylphenol, subsequently forming stable VPAs. It also improves the colour stability of fruit wines and prevents the formation of unwanted off-flavour compounds by not facilitating the reduction of vinylphenol to ethylphenol. As a result, the identification of strains demonstrating elevated HCDC activity constitutes a critical research focus that seeks to improve the coloration of fruit wines, and it presents considerable potential for practical applications. In practical application, the following points should be noted: (1) It is important to assess not only the fermentation capabilities of the yeast strains themselves, but also the adaptability of the HCDC produced by these strains to the fermentation environment during the screening process. (2) In practical applications, enzyme treatments, such as CE, or the incorporation of yeast extract can be employed to enhance the quality of wine. In addition, future research may focus on the optimisation of the fermentation process to further improve the overall quality of the wine. (3) The incorporation of HCA prior to fermentation may enhance the significance of the HCDC effect; however, it is essential to determine the optimal quantity and specific type of HCA to be utilized. The effects of HCDC-expressing yeasts in different systems and fermentation conditions must also be investigated. Future investigations may delve into the optimization of yeast performance via genetic engineering and alternative methodologies to attain enhanced and more uniform color enhancement.
This review was supported by the National Key R&D Program of China (2022YFF1100202 and 2016YFD0400500) and the Science and Technology Project in Beijing (Z201100008920003).
-
The authors confirm contribution to the paper as follows: conceptualization: Peng Y, Huang W, You Y; visualization: Peng Y, Xie Y; formal analysis, investigation: Peng Y, Zhou H; writing – original draft: Peng Y; writing – review and editing: Zhou F, Zhan J, Huang W, You Y; funding acquisition: You Y. All authors reviewed the results and approved the final version of the manuscript.
-
Data sharing not applicable to this article as no datasets were generated or analyzed during the current study.
-
The authors declare that they have no conflict of interest.
- Copyright: © 2025 by the author(s). Published by Maximum Academic Press on behalf of China Agricultural University, Zhejiang University and Shenyang Agricultural University. This article is an open access article distributed under Creative Commons Attribution License (CC BY 4.0), visit https://creativecommons.org/licenses/by/4.0/.
-
About this article
Cite this article
Peng Y, Xie Y, Zhou H, Zhou F, Zhan J, et al. 2025. Hydroxycinnamic acid decarboxylase activity of yeast and its effect on the quality of fruit wines. Food Innovation and Advances 4(1): 127−137 doi: 10.48130/fia-0025-0013
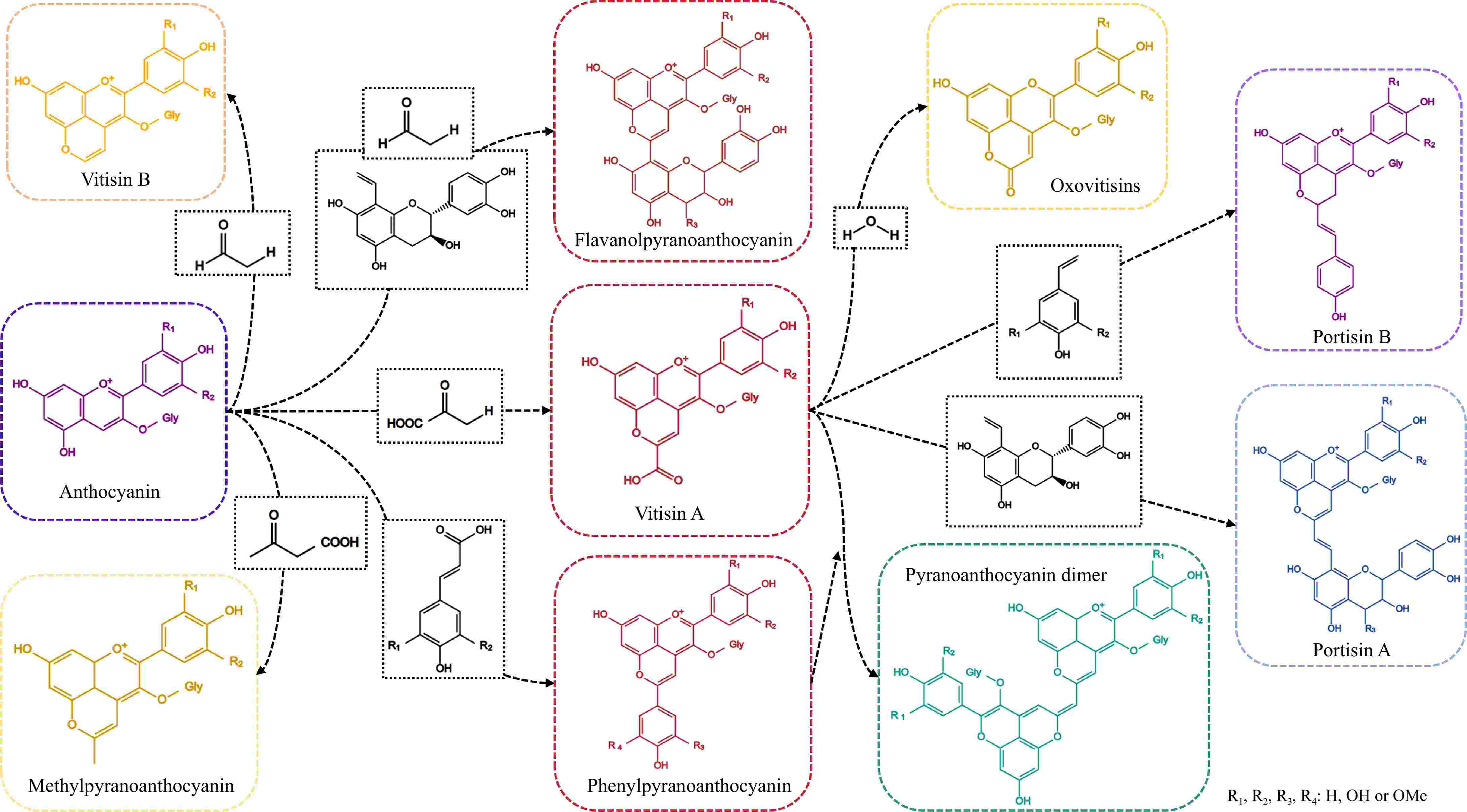