-
Heterosis refers to the phenomenon where the offspring produced by the hybridization of two inbred lines have better adaptability, higher yield, and better tolerance to abiotic and biotic stress compared to the parent line. To date, the yields of maize (Zea mays L.), rice (Oryza sativa L.), rapeseed (Brassica napus L.) and other crops have benefited strongly from the utilization of heterosis through the development of hybrids. In the process of producing hybrid seeds, cross-pollination of parents with genetic diversity is an important step. However, for self-pollinating crops such as rice, this process requires time-consuming emasculation to prevent self-pollination, thus hindering the efficiency of large-scale seed production. One option is to remove the anther or emasculate the anther using machines, manual labor, or chemical treatments, all of which are inefficient, costly, and even unfriendly to the environment. Another option is to use male sterile lines, which are naturally occurring mutants that fail to produce functional pollen and viable male gametes. The development of the tapetum, meiocytes or microspores may be affected in most male sterility mutants, leading to pollen abortion or sterility without pollen. Therefore, plant male sterility is widely used in the context of heterosis because it facilitates hybrid seed production, which contributes greatly to increasing the productivity of many crops worldwide.
Generally, two types of male sterility systems are frequently used in crop breeding: cytoplasmic male sterility (CMS) and genic male sterile (GMS) systems. Cytoplasmic male sterility, which is induced by mutations in cytoplasmic genes, is a maternally inherited phenomenon characterized by a lack of functional pollen. This phenomenon has been documented in more than 200 species of higher plants[1]. The mitochondrial genome of CMS plants is different from that of normal plants, and CMS exhibits maternal inheritance. CMS is always associated with mitochondrial mutations and chimeric open reading frames (ORFs) that are thought to originate from mitochondrial genomic rearrangement[1−3]. The phenomenon of CMS can be inhibited or counteracted by the nuclear-encoded genes known as restorers of fertility (Rfs). Based on the CMS/Rf system, a three-line system of hybrid crop varieties consisting of a male sterile line, a maintainer line and a restorer line is widely used in the commercial production of hybrid seeds (Fig. 1a). The CMS/Rf system also provides an excellent model for studying mitochondrial-nuclear interactions and coevolution in plants.
Figure 1.
The genetic and mechanistic models of cytoplasmic male sterility and genic male sterility. (a) A CMS line contains a sterile cytoplasm and a nonfunctional restorer of the fertility gene (rf), which can be maintained by recurrent crossing to a maintainer line with normal cytoplasm. A restorer line with normal or sterile cytoplasm and a functional restorer gene (Rf) can restore fertility in the F1 hybrid. Mit: Mitochondrion, Nu: Nucleus. (b) The application and molecular mechanism of genes related to environmental male sterility in a two-line hybrid system in rice. The fertility of the EGMS line could switch based on the state of the environment to produce hybrid seeds in a two-line system. The EGMS line acts as the maternal parent to produce F1 hybrid seeds when it is sterile under restrictive conditions and does self-breeding when it is fertile under permissive conditions. (c) A DGMS line was regulated by a single gene/locus and exhibited male sterility under heterozygous conditions. When crossed with fertile plants, the progeny had a 1:1 ratio of sterile to fertile individuals, and the sterile plants could be repeatedly used as DGMS lines.
GMS is caused by mutations in nuclear genes and includes dominant and recessive nuclear sterility. The genetic resources of dominant male sterility in plants provide valuable breeding tools for germplasm innovation and hybrid crop production. However, there are few reports on dominant male sterility in crops. Most GMS lines in use exhibit environment-sensitive genic male sterility (EGMS) these lines represent a typical example of plant reproductive development affected by environmental signals and might be affected by photoperiod, temperature, or humidity. EGMS could be sterile under restrictive conditions, but the fertility could recover under permissive conditions. This characteristic is used in hybrid seed production of two-line hybrid rice, as the EGMS line can cross with restorer lines for seed production when it is sterile and to reproduce itself when it is fertile. Both three-line and two-line systems have been extensively used in hybrid breeding of major crop species for several decades.
-
Plant mitochondrion are semiautonomous organelles that contain their own genome, with highly variable structure and size. Most reported CMS genes are cytotoxic and encode small transmembrane proteins with a size of 10−35 kDa. During microspore development, toxic proteins encoded by CMS genes often alter mitochondrial function in sporophytes or gametophytes, such as ATP synthesis and abnormal programmed cell death (PCD), ultimately leading to male sterility in plants[1].
Numerous studies have shown that CMS related genes are not only caused by SNPs and INDELs, but also attributed to rearrangement and recombination of the mitochondrial genome[1−3]. The sequences obtained through recombination in mitochondria usually contain a portion of known mitochondrial gene sequences and fuse with mitochondrial promoters to form functional genes. For example, in the CMS-WA male sterile lines, the mitochondrial gene WA352 contains three fragments derived from speculated mitochondrial ORFs orf284, orf224, and orf288, as well as a short sequence of unknown origin[4] (Fig. 2a). The chimeric gene WA352, encoding a transmembrane protein, accumulates in the tapetum of anthers at the microspore mother cell stage[4]. WA352 accumulates in the tapetum of pollen mother cells stage and interacts with COX11, thereby inhibiting the function of COX11 in peroxide metabolism and triggering premature tapetal programmed cell death and consequent pollen abortion[4]. Noticeably, the orf352 gene for CMS-RT102 is a variant of WA352 for CMS-WA and shows four amino acid substitutions with the WA352 protein, suggesting that these two CMS systems might share a common ancestor[4,5].
Figure 2.
The CMS genes, restorer genes and mechanistic models of cytoplasmic male sterility in rice. (a) The mitochondrial genes WA352, orf79, orfH79 and FA182 are expressed in CMS lines, damaging the development of anthers and pollen, and are suppressed by the corresponding nuclear Rf genes in hybrids, thereby restoring male fertility. Most Rf genes encode a PPR protein. Rf4, Rf1a, Rf1b, Rf6 and OsRf19 contain 18, 18, 11, 20, and 18 PPR domains, respectively. (b) Proposed model illustrating the mechanism of CMS and fertility restoration in rice. CMS genes derived from the mitochondrial genome are transcribed and translated into proteins with transmembrane domains in mitochondria, leading to an explosion of reactive oxygen species (ROS) and ultimately premature programmed cell death (PCD), resulting in sterile male gametes in CMS lines. Restorer genes derived from the nuclear genome encode proteins with mitochondrial transpeptides in the F1 generation. The restorer proteins translocate into mitochondria and bind to CMS transcripts directly or indirectly to cleave or degrade RNA; alternatively, the interactions between restorer proteins and RNA block the translation of CMS proteins. Therefore, CMS products are eliminated, and male gametes become fertile. (c) The relative positions of the restorer genes Rf4, Rf1a, Rf1b, and OsRf19 on chromosome 10 in different rice varieties. The dashed line represents the unknown sequence in Jinhui3.
In the CMS-BT lines, the male sterility gene orf79 is located downstream of an extra copy of the atp6 gene (B-atp6) in the mitochondrial genome[6]. Thus, orf79 is constitutively cotranscribed with B-atp6 to produce a chimeric transmembrane protein that accumulates mainly in microspores, inducing gametophyte abortion in CMS-BT rice[6,7]. Similarly, the male sterility gene orfH79 in CMS-HL rice is also located downstream of the mitochondrial atp6 gene and shares high nucleotide sequence similarity (98%) with orf79[7,8]. In CMS-HL lines, ORFH79 interacts with subunit P61 of mitochondrial electron transport chain complex III, reducing the enzyme activity of mitochondrial complex III, leading to an increase in reactive oxygen species content and a decrease in ATP concentration, thereby inducing the abortion of pollen[7,8]. In addition, the CDS of L-orf79 in CMS-LD contains one nucleotide change compared with that of orf79 in CMS-BT, which leads to one amino acid substitution[9,10].
The Fujian Abortive (CMS-FA) line was bred from the cytoplasm of Fujian wild rice in China[11−14]. CMS-FA is sporophytic with a no/less-pollen-grain phenotype. To identify the gene that causes CMS traits in the CMS-FA line, the mitochondrial genome sequence of the CMS-FA sterile line was compared to that of the restorer line, the CMS-RT98 sterile line and the maintainer line. Two highly diverged regions were specifically found in the mitochondrial genome of the CMS-FA sterile line, among which located the chimeric gene FA182 based on comparative sequence analysis[12]. Overexpressing FA182 causes male sterility in rice. FA182 consists of three recombinant fragments, a 421-bp fragment that is identical to the Sorghum bicolor mitochondrial sequence (with a nucleotide similarity of 79%), a 71-bp fragment that is identical to the rice mitochondrial sequence, and a 57-bp fragment of unknown origin[12] (Fig. 2a). FA182 is identical to the orf182 gene in CMS-D1 rice, which was identified from wild rice (Oryza rufipogon L.) in Dongxiang, Jiangxi Province, China[12,15].
Many other ORFs have also been identified in CMS rice. Of these, the CW-orf307 gene for CMS-CW encodes a hypothetical protein that contains the 5’ sequence of orf288 and an origin-unknown sequence[16,17]. The orf113 gene of CMS-RT98 encodes a hypothetical protein whose 5’ region is identical to that of nad9, an NADH dehydrogenase subunit 9[18]. WA314 is another CMS gene of CMS-WA, which has been shown to cause partial male sterility in rice through genetic transformation. There are 19 single nucleotide polymorphism (SNP) differences between WA314 and orf314[19]. The orf312 gene in CMS-TA rice is similar to the orf288 gene in Nipponbare and shows differential expression patterns between CMS lines and restore lines[20,21].
Taken together, 10 CMS genes have been identified in rice, most of which were derived from mitochondrial gene rearrangement[1,22]. For example, orf79 for CMS-BT and orfH79 for CMS-HL in rice contained a sequence in the N-terminus similar to that of cox1 and a sequence of unknown origin. WA352 for CMS-WA and orf352 for CMS-RT102 include three fragments derived from putative mitochondrial ORFs, orf284, orf224 and orf288, and a short sequence of unknown origin. In addition, most CMS genes, such as orf79, orfH79, WA352, orf352 and orf312, encode proteins with transmembrane domains. These transmembrane proteins often have a hydrophobic region that is typical of cytotoxic proteins, which may lead to mitochondrial dysfunction in anther sporophyte or gametophyte cells, or increase in reactive oxygen species (ROS), ultimately resulting in programmed cell premature death, leading to male gametes being infertile in CMS lines (Fig. 2b).
Genetic basis of restorer genes in rice
-
The plant mitochondrial genome contains approximately 60 known genes; however, proteomic analysis shows that plant mitochondria contain more than 1,000 proteins, with a small portion encoded by the mitochondrial genome and the majority encoded by the nuclear genome[23,24]. Many studies have shown that mitochondrion-localized nuclear genes, including Rf genes, play important roles in regulating the functions of mitochondrial genes. The harmful effects of the CMS protein on tapetum and pollen development can be rescued through various mechanisms of interaction between the CMS and Rf genes. To date, seven Rfs have been cloned and characterized as pentatricopeptide repeat (PPR) proteins in rice, including Rf1a, Rf1b, Rf5, Rf6, Rf4, OsRf19, and Rf20[6,12,25−30] (Table 1). In addition to PPR proteins, other types of Rfs, including Rf2 and Rf17, have also been identified in rice[10,31].
Table 1. Types and characteristics of CMS/Rf systems in rice.
CMS type Cytoplasm source CMS gene Rf gene Location Ref. CMS-BT O. sativa ssp. indica (Chinsurah Boro II) orf79 Rf1a Chr.10 [6] Rf1b Chr.10 [7] CMS-CW O. rufipogon (W1) CW-orf307 Rf17 Chr.4 [31] CMS-D1 O. rufipogon (Dongxiang wild rice) orf182 UK UK [15] CMS-FA O. rufipogon (Fujian wild abortive) FA182 OsRf19 Chr.10 [12] CMS-HL O. rufipogon (Hong Lian wild rice) orfH79 Rf5 Chr.10 [8,26] Rf6 Chr.8 [29] CMS-LD O. sativa ssp. indica (Lead rice) L-orf79 Rf2 Chr.2 [9,10] CMS-RT98 O. rufipogon (W1109) orf113 Rf98 Chr.10 [37] CMS-RT102 O. rufipogon (W1125) orf352 UK UK [5] CMS-TA O. sativa ssp. indica (Tadukan) orf312 UK UK [20,21] CMS-WA O. rufipogon (Wild abortive) WA352 Rf3 Chr.1 [4] Rf4 Chr.10 [28] Rf20 Chr.1 [30] UK: unknown gene/location. The initial research suggested that the single locus Rf-1 could restore fertility in CMS-BT type sterile lines. Further study revealed that the Rf-1 locus comprises two Rf genes, Rf1a and Rf1b, within a 105 kb region on chromosome 10. Rf1a and Rf1b encode PPR proteins of 791 and 506 amino acids (aa) respectively. Both proteins are located in mitochondria and have a 70% similarity in their protein sequences[25]. The fertility restorer genes Rf5 and Rf6 of CMS-HL are located on chromosomes 10 and 8 of rice, respectively[26,29]. The Rf5 gene is identical to the Rf1a gene of CMS-BT, encoding a protein located in the mitochondria and containing 791 amino acids. Rf6 encodes a mitochondrial-localized PPR protein of 894 amino acids that contains 21 PPR motifs. The fertility of CMS-WA lines can be restored by two dominant Rf genes, Rf3 on chromosome 1 and Rf4 on chromosome 10[4,28]. Rf4 encodes a 782 amino acid mitochondrial localized PPR protein located within the PPR cluster near Rf1a on the chromosome 10 of rice (Fig. 2c). Rf20 is a new fertility restorer gene for CMS-WA that encodes a 440 amino acids protein. Rf20 could restore pollen fertility in some WA-type CMS lines under high temperatures[30]. The fertility restore gene OsRf19 of CMS-FA was identified in Fujian wild rice and mapped to chromosome 10, which encodes a mitochondrial-localized PPR protein of 791 aa that is also close to Rf1a (Fig. 2c). Notably, the sterility-restoration of CMS-BT, CMS-HL and CMS-WA requires two restorer genes. However, the sterility-restoration in the CMS-FA/Rf system is presented as a single-locus inheritance[12,14], which is quite different from the two-locus inheritance of the other CMS systems. This characteristic makes CMS-FA/Rf system more suitable for hybrid rice breeding than the currently widely used CMS systems[12].
Molecular basis of restorer genes in rice
-
Nuclear-encoded restorer of fertility genes rescue the fertility of CMS lines by altering or suppressing the expression of CMS-associated ORFs at the posttranscriptional or posttranslational level[1]. Most fertility restorer genes encode PPR proteins, which have RNA binding activity and are related to the post transcriptional regulation of gene expression in organelles (chloroplasts and mitochondria), including RNA editing, RNA splicing, RNA stabilization, initial translation and other processes[32−34].
RF1A and RF1B process the orf79 transcript through different mechanisms to restore the fertility of CMS-BT rice[25]. RF1A mediates the endonucleolytic cleavage of B-atp6-orf79 mRNA at three major regions; this cleavage interrupts the translation of the orf79 mRNA fragment. However, RF1B mediates the degradation of the B-atp6-orf79 transcript. In the presence of both restorers, RF1A was epistatic to RF1B in the processing of orf79 mRNA[25].
RF5 was unable to directly bind the atp6-orfH79 mRNA in CMS-HL rice. However, RF5 interacts with the glycine-rich protein GRP162 to form a fertility restoration complex, which recognizes and binds to the transcript of atp6-orfH79 and cleaves at nucleotide 1169 of atp6-orfH79 mRNA[26]. RF6 interacts with hexokinase 6 (OsHXK6) to form a complex and binds to the transcript of atp6-orfH79, mediating the cleavage of the atp6-orfH79 mRNA at nucleotide 1238. The duplication of PPR motifs 3–5 is necessary for the fertility restoration function of RF6[29]. Rf5 and Rf6 can restore the fertility of rice CMS-BT, and Rf1a in CMS-BT can also restore the fertility of CMS-HL[35], suggesting that a conserved restoration mechanism may have been established in different CMS lines before domestication.
RF4 can reduce the abundance of WA352 transcripts by 80% and thus restore the fertility of CMS-WA lines[4,28]. However, the amount of the WA352 transcript was not affected by Rf3; instead, the WA352 protein was undetectable in Rf3-restored plants, suggesting that Rf3 probably functions posttranslationally[4]. The PPR protein encoded by Rf20 interacts with the COX11 protein to competitively inhibit the binding of WA352 to COX11, thereby restoring the reactive oxygen species scavenging function of COX11 and partially restoring the fertility of CMS-WA[30].
OsRF19 affects the mRNA expression of FA182 in CMS-FA. RNA analysis revealed that FA182 mRNA was cleaved in the restorer line 9311(FA)R (containing FA182 and OsRf19). OsRF19 reduced the transcript level of FA182 in the restorer line 9311(FA)R by 3.3 times in roots, 11.5 times in stems, 8.2 times in leaves, 6.7 times in panicles, and 8.5 times in anthers compared with that in the CMS-FA line 9311(FA)A (containing FA182). Therefore, OsRF19 restores the fertility of CMS-FA by cleaving and reducing the number of FA182 transcripts[12].
The fertility restorer gene Rf2 for CMS-LD encodes a glycine-rich protein consisting of 152 amino acids and is located in mitochondria[10,36]. The Rf2 gene restores fertility by promoting the degradation of atp6-orf79 mRNA in CMS-LD and CMS-BT rice[10,36]. The fertility restorer gene of CMS-CW is Rf17, a reduced expression allele of the RMS (RETROGRADE-REGULATED MALE STERILITY) gene encoding a mitochondrial targeting protein with 178 amino acids[31]. There is a nucleotide mutation at 2,286 bp upstream of the Rf17 and rf17 start codons, resulting in a change in gene expression levels. Reducing the expression of RMS can restore the fertility of CMS-CW[31]. The Rf98 gene for CMS-RT98 encodes a 762-amino acid protein with 18 PPR motifs, which is responsible for the partial restoration of fertility. The seed setting rate of transgenic plants carrying Rf98 is very low (about 9.3%), and some other genes near the Rf98 locus may be necessary for the full recovery of seed setting[37].
In summary, most Rf genes may restore pollen fertility by affecting the RNA of CMS genes, as restorer proteins translocate into mitochondria and bind to CMS transcripts directly or indirectly to cleave or degrade RNA. Alternatively, the interactions between restorer proteins and the RNA of the CMS gene block the translation of CMS proteins (Fig. 2b). Therefore, CMS products are eliminated, enabling normal development of male gametes.
Evolution of restorer genes in rice
-
Most Rf genes in plants encode PPR proteins with high sequence similarity, typically appearing in clusters. Studies have shown that these clusters may be formed through tandem replication[38,39]. For example, a PPR gene cluster located in an approximately 400-kb region on chromosome 10 of the rice genome consists of at least ten PPR genes, including Rf1a and Rf1b of CMS-BT, Rf5 of CMS-HL, Rf4 of CMS-WA, and OsRf19 of CMS-FA[6,7,12,26−29,39].
Genome sequence analysis revealed 69 haplotypes at the Rf4 locus through structural and copy number variations in the Oryza genus[39]. Structural variation was detected at the Rf4 locus, three PPR genes were detected in rice varieties such as Zhenshan97B and Nipponbare, and seven PPR genes were found in rice varieties such as Minghui63 and Shuhui498. Further research showed that Minghui63 carried two copies of Rf4, Rf4a, and Rf4b, within the 74.8 kb region. In the wild rice O. meyeriana (GG-genome), only a single putative Rf4 variant existed at the Rf4a site; this variant represents the primitive sequence of ancestral Rf4 and evolved various haplotypes in other wild rice species along with sequence variation and genetic recombination. Subsequently, during natural and human selection processes, eight Rf4 haplotypes (H1-H8) were enriched in modern rice varieties. In particular, a haplotype with two copies of Rf4 is widely used in three-line hybrid rice breeding, and nonfunctional rf4i is preferentially selected for breeding the current CMS-WA line[39].
Another interesting case is the evolutionary trajectory of OsRf19. The copy number of PPRs in the OsRf19 region differed greatly among Oryza species[12]. One PPR copy was detected in L. perrieri of the OsRf19 homologous region, and two PPR copies, PPR830 and ORF4, were detected in the most recent common ancestor of the AA and BB lineages of Oryza. Subsequently, independent duplication events occurred in these two ancestral PPR copies. The restorer gene OsRf19 might have originated from an adjacent PPR gene resulting from a recent duplication of the wild relatives of Asian cultivated rice. The orthologue of a PPR gene in this lineage gained the ability to restore the expression of the restorer gene Rf1a/Rf5 in Boro II and Hong-Lian CMS. These results suggested that the fertility restorer genes OsRf19 and Rf1a/Rf5 originated from a common ancestor in ancient times[12].
-
The CMS/Rf system, which consists of the CMS line, the maintainer line, and the restorer line, is a useful tool in three-line hybrid rice production. The CMS line is used as the female parent with male-sterile cytoplasm caused by a CMS gene. The maintainer line contains the same nuclear genome as the CMS line but has a normal fertile cytoplasm. The seeds of the CMS line were obtained by crossing the maintainer line with the CMS line. The restorer lines have one or more functional Rf genes, usually used as the male parent to hybridize with CMS lines to produce F1 hybrid seeds (Fig. 1a).
The first CMS-BT line was developed using the cytoplasm of the indica variety Chinsurah Bolo II and the genetic background of the japonica variety Taichung 65 in 1968 in Japan[6,40]. The BT cytoplasm in combination with its restorer genes, Rf1a and Rf1b, has been widely used for commercial seed production of japonica hybrid rice. The CMS-BT line Taichung 65 was first introduced from Japan by the Chinese Academy of Agricultural Sciences and the Liaoning Academy of Agricultural Sciences in 1972. Since the approval of the first japonica hybrid rice variety, Liyou 57, in 1980, more than 396 varieties, most of which are BT cytoplasm, have been approved for use in China[41]. Japonica hybrid rice is mainly planted in Zhejiang, Jiangsu, Anhui, Liaoning, Hubei, and Yunnan provinces in China. However, due to the limited yield of seed production, not too high heterosis between the parents and less adaptability of japonica hybrid rice, the promotion area of japonica hybrid rice contributes to less than 5% total japonica planting area in China[41,42].
In 1970, a CMS-WA line was first discovered by Longping Yuan in wild rice (Oryza rufipogon) in China. In 1973, the cytoplasmic male sterile line, maintainer line, and restorer line were developed by Chinese breeders, which promoted the development of hybrid rice in China. In 1980, using a combination of Minghui63 and Zhenshan97, Huaan Xie developed an elite hybrid rice variety, Shanyou63, which has the largest cumulative planting area in China[43,44]. Currently, hundreds of CMS-WA lines have been developed and are used in rice production, and the CMS-WA hybrid rice is the most widely used three-line hybrid rice in China[43].
In 1972, the Chinese breeder Yingguo Zhu discovered a male sterility plant among the hybrid offspring of Hongmang wild rice and Liantangzao. Using Liantangzao for multiple generations of backcrossing, a CMS-HL cytoplasmic male sterile line was obtained in 1974. Subsequently, a series of CMS-HL lines, such as YuetaiA, Luohong3A, and Luohong4A, and hybrid rice varieties, such as Honglianyou6, Luoyou8, and Yueyou9, were developed. Currently, HL-type hybrid rice is widely planted in China and Southeast Asian countries.
The CMS-FA line was developed by Naiyuan Wang in the 2000s using the cytoplasm of common wild rice (Oryza rufipogon L.) found in Fujian Province, China. The male sterile lines of CMS-FA are sporophytic with no/fewer pollen grains and thus exhibit complete male sterility; however, the male sterility of the CMS-BT lines and CMS-HL lines is genetically controlled by gametophytic tissue, the fertility of which is easily influenced by the environment. Compared with their WA counterparts, the single restorer gene OsRf19 adequately restored the male fertility of the hybrids but had no negative effects on hybrid performance. Single gene inheritance greatly simplifies the breeding process of restorer lines and greatly accelerates the development of hybrid rice breeding. A series of CMS-FA lines, such as Jinnong2A and Jinnong3A, and hybrid rice varieties, such as Jinnong2you3 and Jinnong3you3, were developed. Hybrids from the CMS-FA/OsRf19 system showed excellent performance compared with the corresponding controls, and a number of FA hybrids have been bred and will be released for commercial use. Therefore, the CMS-FA/OsRf19 system is a promising alternative for hybrid rice breeding[12].
-
EGMS refers to a special class of genic male sterility and allows for the reversibility of male fertility in response to a particular environment. EGMS is feasible for the breeding of two-line hybrids in a range of crops[45−47]; here, the focus is on rice (Fig. 1b). The starting point for EGMS in rice was the discovery of a natural mutant line called Nongken 58S in 1973[48], which shows photoperiod-sensitive genic male sterility (PGMS). This mutant is sterile under long-day conditions (restrictive conditions) and can serve as a male-sterile line for hybrid seed production, and fertile under short-day conditions (permissive conditions) and can propagate, and this characteristic has started the journey of hybrid rice breeding with two-line system in China (Fig. 1b). Since then, a number of genes responsible for EGMS have been cloned and investigated (Table 2), such as pms1[49−51], pms3[50], and csa2[52] for PGMS; csa for reverse PGMS[52−54]; tms5[55], ugp1[56], tms10[57], ostms15[58], ostms18[59], ostms19[60], and tms9-1/OsMS1wenmin1[61] for temperature-sensitive genic male sterility (TGMS); and ososc12/ospts1[62], osgl1-4/oscer1[63], hms1 and hms1i[64] for humidity-sensitive genic male sterility (HGMS) in rice (Table 2). The exploration of the related molecular mechanisms of EGMS genes and their responses to diverse environmental factors greatly facilitates the development of two-line hybrid rice.
Table 2. Types and characteristics of EGMS genes cloned in rice.
EGMS line EGMS type Locus Encoding product Biological pythay Ref. NK58S PGMS pms3 Long noncoding RNALDMAR Noncoding RNA and RNA processing [49,50] NK58S PGMS pms1 Long noncoding RNA PMS1T Noncoding RNA and RNA processing [51] csa mutant rPGMS csa R2R3 MYB transcription factor Sugar distribution [52−54] csa2 mutant PGMS csa2 R2R3 MYB transcription factor Sugar distribution [52] AnnongS-1 TGMS tms5 RNase Zs1, 2’3’-cyclic phosphatase of tRNA RNA processing [55,78] ugp1mutant TGMS ugp1 UDP-Glucose Pyrophosphorylase1 RNA processing [56] tms10 mutant TGMS tms10 A leucine-rich receptor kinase LRR-RLK Signaling transduction [57] Hengnong S-1, Tian1S TGMS tms9-1/OsMS1wenmin1 PHD zinc finger protein Pollen developent [61,85,86] Peiai64S TGMS p/tms12-1 Small RNA Noncoding RNA [69] ostms18 mutant TGMS ostms18 GMC oxidoreductase Pollen wall formation [59] ostms15 mutant TGMS ostms15 A leucine-rich receptor kinase LRR-RLK Pollen developent [58] ostms19 mutant TGMS ostms19 PPR protein ROS accumulation [60] E157, S1708 and S4928 HGMS ososc12/ospts1 Bicyclic triterpenoid polyacetylacetone synthase Pollen coat [62] hms1 mutant HGMS hms1 3-β-ketoacyl-CoA synthase 6 Pollen coat [64] hmsli mutant HGMS hmsli Very-long-chain enoyl-CoA reductase Pollen coat [64] osgl1-4 mutant HGMS osgl1-4/oscer1 Acyl-CoA synthetase Pollen coat [94] ago1d mutant TGMS ago1d Argonaute protein PhasiRNA production [77] Noncoding RNA and RNA processing play important roles in EGMS
-
Long noncoding RNAs (lncRNAs) or small RNAs provided the first insight into the control of EGMS. Two lncRNAs, LDMAR, and PMS1T, which are encoded by pms3 and pms1, respectively; pms3, which is involved in fertility segregation between Nongken 58S (58S) and its wild-type parent Nongken58 (58N); and pms1, which is involved in fertility segregation between Nongken 58S and other varieties[49,51], promote the understanding of RNA processing in photoperiod-sensitive male sterility (Fig. 3a).
Figure 3.
Noncoding RNAs and RNA processing play important roles in EGMS. (a) The molecular mechanism of photoperiod-sensitive male sterility regulated by noncoding RNAs produced from the pms3/ptms12-1 and pms1 loci. HT, high temperature; LD, long day. (b) The molecular mechanism of thermosensitive male sterility regulation through RNA processing in tms5 and ugp1. The mutation in tms5 leads to a short version of the tms5 protein, which fails to process the mRNA of UbL40 under HT and results in male sterility. (c) The molecular mechanism of thermosensitive male sterility regulation through RNA processing of ugp1. More primary transcripts containing introns accumulated in ugp1 than in WT, and the introns could not be spliced under HT, which caused no UGP1 protein to maintain normal pollen development in ugp1, resulting in male sterility under HT. LT, low temperature.
The single C-G SNP contained in the long-day-specific male fertility-associated RNA (LDMAR) encoded by the pms3 locus is co-segregated with the phenotype of PGMS[65−68]. Why can a single SNP of the LDMAR strongly change fertility in response to day length? Under long-day conditions, a relatively high expression level of this lncRNA is necessary for normal pollen development in fertile rice plants[49,50]. However, the C to G mutation would change the secondary structure of LDMAR, and the 21-nt sRNA psi-LDMAR generated from the promoter of LDAMR may increase the methylation level in the promoter region; this would decrease the expression level of LDMAR expression specifically under long days, which leads to premature programmed death of the tapetum and ultimately PGMS. Interestingly, the same locus, P/TMS12-1, mediating temperature-sensitive male sterility was cloned from the indica TGMS line Peiai64S[69]. P/TMS12-1 could be processed to the 21-nt small RNA osa‐smR5864 with the same C-G SNP. This change in osa‐smR5864m in the TGMS line affects the expression of the target genes and confers TGMS in indica rice. Therefore, the same gene, pms3, could regulate both PGMS and TGMS in different backgrounds, and noncoding RNAs play important roles in both types of EGMS (Fig. 3a).
The other PGMS locus cloned from 58S is pms1, which also encodes the lncRNA RNA PMS1T[51,68,70,71]. PMS1T can generate 21-nt phasiRNAs triggered after being cleaved by the 22-nt microRNA miR2118. The abundance of phasiRNAs produced is negatively correlated with fertility, and the highest accumulation of phasiRNAs occurred in the sterile 58S strain under long days. The presence of the only functional SNP S2 G to A located near the miR2118 target site may affect the shear efficiency, resulting in increased accumulation of phasiRNAs in the PGMS line under long days[51] (Fig. 3a).
PhasiRNAs are widely present in the inflorescence tissues of grasses and play important roles in male reproductive development[72−75]. Mutations in several genes involved in phasiRNA synthesis are associated with environmentally sensitive male sterility. For example, the dcl5 mutant in maize was sterile under high temperature but fertile under low temperature[76]. The ago1d mutate in rice also showed sterility under high temperature but fertility under low temperature[77].
In addition to long noncoding RNAs and phasiRNAs, temperature-sensitive RNA splicing and accumulation are also involved in the TGMS of TMS5[55] (Fig. 3b) and UGP1[56] (Fig. 3c), which further supports the important roles of RNA in plant adaptation to environmental changes. The TMS5 gene encodes a conserved short version of ribonuclease Z (RNase ZS1). RNase ZS1 could process UbL40 (Ubiquitin-60S ribosomal protein L40) mRNA into multiple fragments in vitro and in vivo in wild-type plants, but the C-to-A mutation at position 71 in the sterile line resulted in the early termination of RNase ZS1. Thus, in the TGMS line, the inability of RNase ZS1 to work at high temperature resulted in the excessive accumulation of UbL40 mRNA, leading to male sterility[55]. A latest research on tms5 revealed that TMS5 has the activity of tRNA 2′,3′-cyclic phosphatase (cP) and mediates tRNA recycling pathway by repairing cP-ΔCCA-tRNAs to replenish the pool of mature tRNAs[78]. At high temperatures, the mutated tms5 impaired the repair activity, leading to more cP-ΔCCA-tRNA accumulation and a decreased pool of mature tRNAs, especially tRNA-Ala, resulting in male sterility. At low temperatures, fewer cP-ΔCCA-tRNAs are generated, either in the wild type or in the tms5 line carrying impaired cP-ΔCCA-tRNA repair activity, and there are sufficient mature tRNAs to maintain the normal development of male reproductive tissues, so the tms5 line is fertile. The fertility of tms5 at high temperatures could be partially restored by the overexpression of tRNA-Alas to add mature tRNAs, or completely restored by the knockout of OsVms1, which is responsible for generating cP-ΔCCA-tRNAs[78]. And the critical sterility-inducing temperature of the tms5-based TGMS line are regulated by two E3 ubiquitin ligase genes, CSIT1[79], and CSIT2[80]. The completely sterile temperature increased from 26 °C in TGMS line AnS-1 to 32 °C in double mutant tms5/csit1 or tms5/csit2. Both CSIT1 and CSIT2 could bind to ribosomes and participate in the ribosome-associated quality control process and can also ubiquitinate anther-related genes. At high temperatures in the TGMS line, the mutation of csit1 or csit2 inhibits the negative effects on the ubiquitination system and protein translation due to the loss of function of tms5, leading to increased accumulation of anther-related proteins, including catalase, to ensure the removal of ROS in anthers and normal PCD, resulting in higher critical sterility-inducing temperature in the tms5-based TGMS lines[79,80]. In addition, OsbHLH138 has been identified to bind to the promoter directly to active the expression of TMS5[81], whereas OsAL5 (OsAlfin Like 5) has been identified to repress its expression by binding to the promoter region[82]. And another transcript factor GATA10 has been identified to activate the expression of UbL40 by binding to its promoter directly and to regulate fertility conversion[83]. The cosuppressing line Co27, which was generated by the overexpression of the UDP-glucose pyrophosphorylase 1 (UGP1) gene in rice, exhibits thermosensitive genetic male sterility[56]. In the Co27 line, there are primary transcripts containing unprocessed introns of UDP1 transcripted from the overexpression construct. These aberrant transcripts of UGP1 cannot be properly spliced at high temperature, resulting in complete inhibition of protein expression and male sterility; some of the aberrant transcripts are normally spliced at low temperature, resulting in the expression level of the UGP1 protein being equivalent to that of the wild type, thus maintaining normal fertility[56].
Gene pairs involved in EGMS
-
Multiple gene pairs, such as pms1 and pms3, csa and csa2, and tms10 and tms10l, are likely involved in conferring EGMS through related pathways (Fig. 4). The relationships among gene pairs and how they are responsible for the EGMS phenotype differ.
Figure 4.
The gene pairs involved in EGMS. Three gene pairs conferring environmentally sensitive male sterility, namely, pms1 and pms3, csa and csa2, and tms10 and tms10l, are listed in the figure. LD, long-day conditions. SD, short-day conditions. LT, low-temperature conditions. HT, high-temperature conditions. (a) Both pms1 and pms3 are required to induce the PTGS trait in 58S. Rice plants lacking either pms3 or pms1 are fertile under both LD and SD. NIL(MH), a near-iso-genic line of pms1 in the background of 58S in which the PMS1 gene was introduced from MH63, was constructed by backcrossing with 58S. (b) The rPTGS csa mutant shows a sterile phenotype under SD, while the PTGS mutant csa2 is semisterile under LD. The double mutant csa/csa2 was sterile under LD and semisterile under SD. (c) tms10 is TGMS and sterile under HT, whereas tms10l is fertile under both HT and LT. The double mutant tms10/tms10l is sterile under both HT and LT.
Both pms1 and pms3 are required to induce PGMS. Rice plants with only pms3 or pms1 remain fertile under both LD and SD conditions, suggesting that lacking any one of these loci would lose the fertility conversion sensitivity to photoperiod[49,51,65,66,68,84] (Fig. 4a).
In addition, the two MYB proteins CSA and CSA2 coregulate sugar transport through differential expression under short-day or long-day conditions. Both genes exhibited a reverse PGMS or PGMS phenotype after mutation, as csa is sterile under short days, and csa2 is semisterile under long days[52,54] (Fig. 4b). The double mutant csa csa2 showed semisterility, as did the single csa2 mutant under long days, and complete sterility, as did the single csa mutant under short days. This indicates that CSA and CSA2 controlled anther development independently in response to photoperiod and may share the same downstream genes, such as MTS8[52,54].
TMS10 has a closely related homologous gene, TMS10-Like (TMS10L). Both genes encode leucine-rich repeat receptor-like kinase (LRR-RLK), which has a complementary relationship with anther development[57] (Fig. 4c). The mutation in TMS10 results in TGMS, and tms10l is fertile at both high and low temperatures, but the double mutant tms10 tms10l shows complete male sterility under both high and low temperatures. TMS10 is crucial for normal anther development under high-temperature conditions, whereas TMS10L functions redundantly with TMS10 under low temperature conditions to ensure male fertility. These findings highlight their vital roles in maintaining the normal function of male reproductive tissues under various temperature conditions.
In summary, multiple EGMS gene pairs conferring photoperiod- and temperature- sensitive sterility through complex mechanisms, potentially driven by their genetic similarities or the ability to share downstream signalling pathways.
Pollen development is closely associated with EGMS
-
Pollen development is finely regulated, and this process is highly sensitive to the environment. Currently, many environmentally sensitive male sterility lines have been found to harbor mutations in pollen development genes. Representative examples include OsMS1wenmin1[61], ostms15[58], ostms18[59], and ostms19[60], as well as all humidity-sensitive male sterility genes related to pollen wall development (Fig. 5).
Figure 5.
Pollen development in relation to EGMS. (a) The molecular pathway of TGMS regulated by OsMS1wenmin1. LT, low-temperature conditions. HT, high-temperature conditions. (b) The mechanisms by which humidity-sensitive male sterility is regulated by OsOSC12/OsPTS1, OsGL1-4/OsCER1, and HMS1-HMS1I. LH, low-humidity condition. HH, high-humidity condition. Due to the reduction in fatty acids in the pollen coat in the HMS line under low humidity, the HMS line fails to finish adhesion and hydration, leading to sterility. However, under high humidity, a defective pollen coat is sufficient to complete adhesion and hydration in the HMS line to maintain male fertility.
OsMS1 encodes a PHD-finger histone binding protein[85], and the L301P mutation resulting from a point mutation from T to C[86] at the LXXLL motif in tms9-1/OsMS1wenmin changes the subcellular localization from the nucleus to both the nucleus and cytoplasm in the TGMS line Hengnong S-1 and Tian1S[61,86]. At low temperatures, both the OsMS1 and OsMS1wenmin proteins interact with TDR in the nucleus to activate target genes, including EAT1. However, under high temperatures, some of the OsMS1wenmin protein is located in the cytoplasm. Therefore, there is not enough nuclear OsMS1wenmin protein to interact with TDR, which led to male sterility[61] (Fig. 5a).
OSTMS15 encodes the same LRR-RLK protein with a cloned gene MSP1[58,87], and its interaction with the ligand OsTDL1A is weakened by the point mutation from Val to Glu in the TIR motif of ostms15, which results in impaired tapetum and sterility under high temperature conditions. While at low temperatures, the protein interaction in the ostms15 mutant is partially restored with slower development, resulting in the production of fertile plants due to the compensation of defective tapetum initiation. Similarly, OsTMS18/OsNP1 encodes a glucosemethanol-choline (GMC) oxidoreductase, and the point mutation from Gly to Ser in ostms18 leads to a defective pollen wall, which results in male fertility under high temperature conditions[59]. At low temperatures, due to its much slower development, the defective pollen wall is sufficient to support the complete development of pollen in the ostms18 mutant. The OSTMS15 and OsTMS18/OsNP1 cases confirmed that fertility conversion in the PGMS and TGMS lines may be attributable to slower development in response to low temperature in rice, which was similar to the findings for rvms, cals5, rpg1, acos5, cyp703a2, and abcg26 in Arabidopsis[88−93].
The ROS-related pathway may coexist with the slow development pathway in the rice PGMS and TGMS lines. OsTMS19 encodes a pentatricopeptide repeat protein (PPR) that is highly expressed in the tapetum and located in mitochondria[60]. In ostms19, the mutated PPR protein caused abnormal mitochondrial function, leading to excessive ROS accumulation in anthers. ROS cannot be scavenged completely under high temperature or long days, resulting in male sterility, whereas ROS accumulation is reduced under low temperatures or short days, thereby resulting in fertility restoration due to the effective removal of ROS in anthers. Notably, the same ROS-related pathway also exists in tms5, ostms15, and ostms18[60]. Therefore, the genes related to these two pathways might be a source for the development of new photosensitive or thermosensitive male sterile rice plants in the future.
In humidity-sensitive genic male sterility lines, the seed setting rate is low under low humidity conditions but returns to normal under high humidity conditions. At present, four genes conferring humidity-sensitive male sterility, ososc12/ospts1[62], osgl1-4/oscer1[63,94], hms1[64], and hms1i[64], have been found in rice, all of which are related to the production of fatty acids in the pollen coat (Fig. 5b). OsOSC12/OsPTS1 encodes a conserved triterpene synthase involved in the biosynthesis of isoprenoids, which constitute pollen coats. In the ososc12 mutant line, three fatty acids were reduced in the pollen coat[62]. OsGL1-4/OsCER1 belongs to the Glossy1 family and is involved in very-long-chain (VLC) alkane biosynthesis. In the osgl1-4/oscer1 mutant line, VLC alkaneswere reduced in the pollen coat[94]. HMS1 encodes a β-ketoacyl-CoA synthase, which, together with its interating gene HMS1I, is involved in the biosynthesis of VLC fatty acids, especially the conversion from C24 to C26/C28. In the hms1 and hms1i mutant line, the C26 and C28 fatty acid contents decreased[64]. The mechanisms of HGMS are mostly focused on the regulation of pollen outer coating contents. A deficiency in the pollen outer coating causes rapid dehydration of the pollen grains, thus affecting the adhesion and germination of the pollen grains on the stigma and leading to sterility under low humidity conditions (Fig. 5b). However, high humidity compensates for this deficiency, and thus, the mutants exhibit fertility at a relative humidity > 80%, which provides an available strategy for future breeding.
EGMS has significant applications in hybrid rice breeding. In recent years, with the advancement of molecular biology and genomics technologies, substantial progress has been made in cloning EGMS genes, understanding their functions, and elucidating their regulatory mechanisms. In the future, the integration of multiomics technologies will reveal the complex regulatory networks of EGMS. Additionally, gene editing technologies such as CRISPR/Cas9 will play a crucial role in the research and application of EGMS systems, enabling targeted modification of key genes to enhance breeding efficiency and stability. Furthermore, as global climate change intensifies, studying the effects of different environmental conditions on EGMS performance has become increasingly important. This will aid in the selection and cultivation of superior rice varieties that can adapt to varying climate conditions, ensuring food security. The construction of comprehensive breeding strategies, such as combining EGMS systems with marker-assisted selection, will further drive innovation and development in rice breeding.
-
Dominant genic male sterility (DGMS) results from mutations in dominant genes within nuclear genomes. The genetic characteristics of DGMS differ from those of CMS and recessive genic male sterility. The DGMS lines maintained a stable sterile phenotype under heterozygous conditions. Progeny with a 1:1 ratio of fertile to sterile individuals are produced by crossing heterozygous sterile plants with fertile plants. The progeny of fertile plants do not further segregate for fertility, while sterile plants can be repeatedly used as sterile lines[1,95] (Fig. 1c).
Genetic resources for DGMS in plants are important breeding assets for the development of novel germplasm and the creation of hybrid crops. Nevertheless, genetic resources for dominant genic male sterility are extremely rare in natural germplasm[95]. To date, there have only been nine cases reported in rice so far, including Pingxiang dominant genic male sterility rice, lower temperature thermosensitive dominant male sterility rice '8987', Sanming dominant genic male sterility rice, japonica dominant genic male sterility rice w450, and OsDMS-2 dominant male sterility rice[96−102]. These genes were detected in both hybrid and inbred populations. Additionally, three dominant genic male sterile lines, zhe9248 M1, Orion 1783, and Kaybonnet 1789, were generated through artificial mutagenesis[103,104], whereas OsDMS-1-dominant male sterile rice was derived from tissue culture[105] (Table 3).
Table 3. The dominant genic male sterile lines and the regulatory genes in rice.
DGMS line Gene (location) Origin Source Ref. Sanming DGMS *SDGMS/OsRIP1 (Chr.8) Hybrid SE21S × Basmati 370 [98,101,108,109] Pingxiang DGMS Ms-P (Chr.10) Hybrid Pingai 58 × Huaye [97,100] 8987 TMS (Chr.6) Hybrid 3304 × Minghui 63 [96] W450 UK Hybrid Japonica × PGMS [102] OsDMS-2 OsDMS-2A (Chr.2), OsDMS-2B (Chr.8) Inbred 8B1390 [99] zhe9248 M1 UK Artificial mutation Zhe 9248 [103] Orion 1783 UK Artificial mutation Orion [104] Kaybonnet 1789 UK Artificial mutation Kaybonnet [104] OsDMS-1 OsDMS-1A (Chr.1), OsDMS-1B (Chr.2), OsDMS-1C (Chr.3) Tissue culture Zhonghua 11 [105] UK indicates an unknown gene/location. * indicates that the gene has been cloned. Dominant genic male sterility regulatory genes cloned from rice
-
In the past decades, transgenic technology has been explored to create DGMS lines by expression of the barnase gene, which is driven by the tapetum specific promoter BoA9 in rice[106]. The EAT1 gene, which is essential for tapetal programmed cell death, expressed by the maize ubiquitin promoter was also used to create transgenic DGMS rice[107]. However, despite some natural germplasms of genetic resources for dominant genic male sterility being obtained, the regulatory genes and their mechanisms in most DGMS lines remain unclear.
Recently, a causal gene in charge of rice DGMS was successfully cloned, for the first time in the Sanming Dominant Genic Male Sterile (SDGMS) Rice. The SDGMS line was first discovered in an F2 population that resulted from a cross between SE21S and Basmati370 by the Sanming Institute of Agricultural Science[98,101]. The SDGMS lines consistently exhibit complete and stable male sterility under varying day lengths and temperatures, making them highly valuable in rice breeding programs. The findings demonstrated that SDGMS rice was caused by a 1978-bp retrotransposon; this retrotransposon spontaneously moved and was inserted whthin the promoter region of the SDGMS gene, which encoding a ribosome-inactivating protein, and activated its specific expression in tapetal cells. SDGMS protein could inhibit protein synthesis at translational level, and the expression of SDGMS triggers a defence response in rice anther, which results in aberrant PCD of tapetal cells during the microspore mother cell stage and a subsequent failure in the development of anther. Furthermore, overexpression of SDGMS and sdgms may improve rice plants resistant to M. oryzae RB22[108]. Another research showed that specific expression of OsRIP1 (SDGMS) in rice roots and leaves result in lethal tissues, and OsRIP1 toxified plant over its translation inhibition activity rather than RNA depurating activity[109].
Dominant genic male sterility regulatory genes cloned from other crops
-
In addition to SDGMS in rice, four DGMS genes have been identified in other crops. In wheat, the orphan gene MS2 was specifically expressed in anther which was caused by the insertion of a retroelement in its promoter area. The MS2 protein could interact with TaRomo1 (wheat ROS modulator 1), promoting its conversion from monomers to inactive oligomers, which reduces ROS signalling in the anther, causing sterility in the Taigu DGMS line[110−112]. In rapeseed, a mutator-like transposable element (MULE) insertion into the second intron of MS5a generates the MS5b allele, which could act as a suppressor of the maintainer allele MS5c and inducing DGMS[113,114]. In maize, a single amino acid mutation in Ms44 causes DGMS by blocking protein processing and inhibiting tapetal cell release of protein into the locule[115]. In cabbage, The binding of the ethylene response factor BoERF1L is affected via a 1-bp deletion within the Ms-cd1 promoter, which increases Ms-cd1 expression and confers DGMS[116]. Taken together, the proteins encoded by these genes and the mechanisms they regulate differ significantly.
Models for the regulation of male sterility by DGMS genes
-
To date, only five genes that regulate DGMS have been identified in crop species. Nonetheless, investigations into these five DGMS lines have shed light on two principal models that cause DGMS. First, the increase in gene dosage caused by gain-of-function mutations could be brought on by transposon insertions or point mutations in the promoter region, which increase gene expression or increase protein dose, disrupting the normal development process of anthers and causing DGMS, as exemplified by rice SDGMS, wheat MS2, and cabbage MS-cd1[108−112,116−118] (Fig. 6a). Second, loss-of-function mutations arise from decreased gene dosage (haploinsufficiency), which results from transposon insertions or point mutations in the coding region leading to protein dysfunction. Loss of function of pivotal proteins could result in anther development arrest and cause DGMS, as observed in rapeseed MS5b and maize MS44[113−115,117,118] (Fig. 6b).
Figure 6.
The mechanistic models of dominant genic male sterility in crops. (a) Gain-of-function caused by a mutation in the promoter region, which increases the expression level of genes. (b) Loss of function due to a mutation in the coding region, which results in protein dysfunction.
Among the five cloned dominant genic male sterility regulatory genes, rice SDGMS, wheat MS2 and rapeseed MS5 are all associated with transposon movement[108,109,111−114]. Organisms possess diverse mechanisms to modulate transposon activity through epigenetic modifications and small RNA pathways. In addition, transposable elements can be activated under stress conditions or during hybridization[119,120]. Notably, a large proportion of DGMS lines in crop species were identified within the progeny of hybrid populations, some of which may be due to the movement of transposable elements[121]. Therefore, current studies in DGMS have underscored the critical role of transposon activity in the emergence of new phenotypes and in the promotion of crop breeding.
Utilization of dominant genic male sterility in crossing and recurrent selection breeding
-
The dominant genic male sterility lines serve as invaluable tools for facilitating outcrossing, an aspect that can be applied in numerous ways within various breeding programs. This application encompasses a range of strategies, including but not restricted to: (1) targeted introgression of a desirable allele for an agronomic trait through successive backcrossing, which enables the swift enhancement of superior cultivars[122]; (2) random introgression of genomic fragments from donor lines by backcrossing to create near isogenic introgressed lines (NIILs), thereby expanding the genetic variation of the breeding lines[123]; and (3) improvement of the cyclic population involved a substantial amount of parental lines by recurrent selection, which can simultaneously enhance multiple traits in a single system, including improved nutrient use efficiency, enhanced stress resistance, and increased yield potential and quality[124]. Therefore, although DGMS lines are not typically directly utilized for the production of hybrid seeds, they can serve as an effective breeding tool for the rapid improvement of parent lines of hybrids. Furthermore, DGMS lines could be developed for any breeding parents, obviating the necessity for manual emasculation, which can significantly increase the efficiency of breeding programs.
-
Rice is one of the most important cereal crops in the world. Hybrid rice has resulted in a substantial increase in food production over the past 50 years and has made a great contribution to safeguarding the food supply in China. However, rice is a self-pollinating crop that is a major barrier to hybrid seed production. Since the 1970s, the discovery and application of rice male sterile lines have broken the technical bottleneck of heterosis utilization in self-pollinating crops[43,125]. Currently, commercial hybrid rice production includes a cytoplasmic male sterile line-based three-line system and a genic male sterile line-based two-line system[1,126]. Since its commercial release in the 1970s, the yield of hybrid rice has increased by approximately 20% compared with that of inbred rice varieties[126]. In recent years, with the development of genetic engineering and molecular biology, third-generation hybrid rice with the genetic engineering of sterile lines as the core technology has emerged, opening up a new direction for the development of hybrid rice technology[127]. The intelligent nuclear sterile line combines molecular design with hybrid breeding, utilizing the common nuclear male sterile genes in crops to create homozygous male sterile lines. Corresponding maintainer lines are created by transferring linked fertility restoration genes, pollen sterility genes, and marker genes into sterile lines, thereby solving the problems of stability and compatibility of sterile lines in the process of hybrid breeding[127]. Currently, the planting area of hybrid rice in China is approximately 17 million ha, and the increase in grain consumption every year can feed 80 million people. China has fed nearly one-fifth of the world’s population with less than 8% of the world’s arable land, making an enormous contribution to world food security, in which hybrid rice plays an indispensable role. Therefore, the cloning, mechanism, and application of rice male sterility and fertility restoration genes are hot topics in plant biology research.
The exploration of CMS and GMS also raises a number of basic biological questions. For example, how do restorer genes interact with CMS genes to restore fertility, and how do mitochondrial genes retrogradely regulate signals to nuclear genomes? What is the perceptual mechanism that mediates the transmission of external environmental signals to internal cellular pathways? The results of these multifaceted studies will pave the way for understanding complex regulatory networks and ultimately lead to a deeper understanding of the mechanisms underlying male sterility and fertility restoration in rice. Understanding the mechanism of male infertility will help us more conveniently utilize male sterility in rice and promote future hybrid rice breeding.
This work was supported by grants from the National Natural Science Foundation of China (31991223 and 32341031), the National Key Research and Development Program of China (2022YFF1002100), the Hubei Key R&D Program (2020BBA034), the Hubei Key R&D Program in Hongshan Lab (2021hszd005, 2022hszd017), the National Key Laboratory of Crop Genetic Improvement Self-research Program (ZK202301), the Fundamental Research Funds for the Central Universities (2662023SKQD001 and 2662023PY002), the Earmarked Fund for China Agriculture Research System (CARS-01), the China Postdoctoral Science Foundation (2021M691181), and the Science and Technology Major Program of Hubei Province (2022ABA001).
-
The authors confirm contribution to the paper as follows: study conception and design: Ouyang Y, Jiang H; data collection: Cai Z, Xu C, Liu X, Lv Y, Ouyang Y, Jiang H; draft manuscript preparation: Cai Z, Xu C, Ouyang Y, Jiang H. All authors reviewed the results and approved the final version of the manuscript.
-
Data sharing not applicable to this article as no datasets were generated or analyzed during the current study.
-
The authors declare that they have no conflict of interest. Yidan Ouyang is the Editorial Board member of Seed Biology who was blinded from reviewing or making decisions on the manuscript. The article was subject to the journal's standard procedures, with peer-review handled independently of this Editorial Board member and the research groups.
-
# Authors contributed equally: Zhaoxia Cai, Conghao Xu
- Copyright: © 2024 by the author(s). Published by Maximum Academic Press on behalf of Hainan Yazhou Bay Seed Laboratory. This article is an open access article distributed under Creative Commons Attribution License (CC BY 4.0), visit https://creativecommons.org/licenses/by/4.0/.
-
About this article
Cite this article
Cai Z, Xu C, Liu X, Lv Y, Ouyang Y, et al. 2024. Exploiting male sterility toward the development of hybrid rice. Seed Biology 3: e019 doi: 10.48130/seedbio-0024-0018
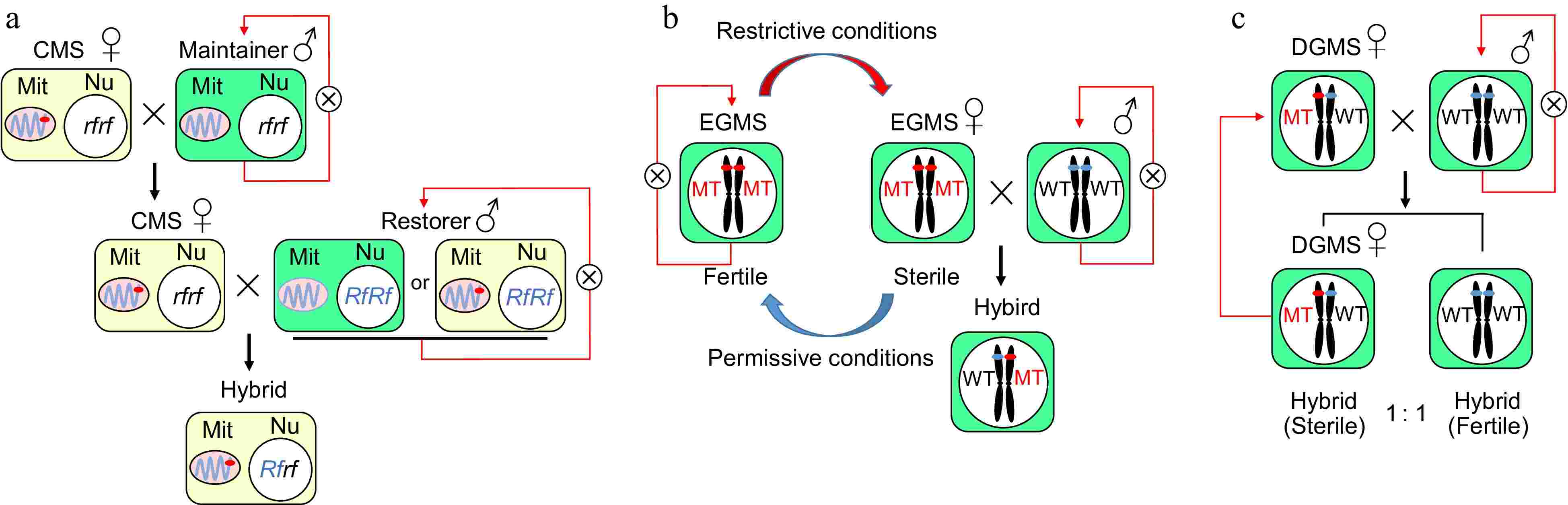