-
Contaminated soil with heavy metals represents a significant environmental concern that has garnered increasing public attention due to its potential impact on agricultural product safety and human well-being[1−3]. Heavy metals, natural elements with the potential for toxicity upon accumulation in soil, are commonly characterized as metallic and metalloid elements with atomic mass greater than 20 and specific gravity exceeding 5, including Cd, Hg, Cu, As, Pb, Cr, Ni, and Zn. From a biological perspective, the term 'heavy' encompasses an array of metals, and in certain cases metalloids, which even at low concentrations, can pose toxicity to plants and animals[4]. The primary sources of heavy metal pollution in soil are predominantly attributed to human activities such as mining, metallurgical processes, agricultural practices (including fertilizer and pesticide usage), improper electronic waste disposal, battery waste, sludge, and mismanagement of materials containing heavy metals[5]. Common heavy metals of concern include Pb, Cd, Hg, As, Cr and Ni. Among these, As, Cd, Cr(VI), Hg, and Pb are classified as non-threshold toxicants capable of inducing toxicity at remarkably low concentrations[6]. Numerous researchers underscore the ecological and human health consequences of heavy metal contamination in soil[7−9]. These metals can disrupt the natural equilibrium of ecosystems by impairing soil microorganisms, diminishing soil fertility, and inhibiting plant growth. Furthermore, they can enter the food chain through plant uptake, resulting in bioaccumulation in animals and posing latent threats to human health. Prolonged exposure to heavy metals can lead to various health issues, including respiratory disorders, cardiovascular diseases, renal impairment, and neurological disorders[10]. Therefore, addressing heavy metal pollution in soil is paramount for safeguarding ecosystems and human health.
Zeolites are naturally occurring aluminosilicate hydrates that exhibit more than 50 distinct forms[11,12] and find a plethora of applications. They serve as soil conditioners, nutrient supplements for both animals and aquatic life, and additionally function as heat storage materials. Moreover, zeolites possess the capacity to serve as adsorbents, ion exchangers, molecular sieves, and catalytic agents in various chemical reactions[13,14]. These applications are mainly based on their ion exchange properties[15].
According to Johnson et al., the structure of zeolites consists of three-dimensional frameworks composed of alumina and silicate tetrahedral units linked together by oxygen atoms. This framework contains interconnected channels and pores, imparting zeolites with distinctive properties such as selective adsorption and molecular sieving[16]. The composition of zeolites can vary, with different ratios of aluminum and silicon determining the framework charge and the type of cations present within the channels. These voids provide zeolites with a large surface area, enabling high adsorption capacity. Furthermore, properties of zeolites, such as thermal stability, acidity, and ion exchange capability, can be tuned by controlling the framework composition and the choice of cations. Additionally, natural zeolites are regarded as beneficial soil amendments, possessing water and nutrient retention abilities. They enhance permeability, saturated hydraulic conductivity, cation exchange capacity, and mitigate deep percolation losses[17−21].
Despite its many advantages, natural zeolite has little or no affinity for anions due to its negative surface charge[22−25]. In the study conducted by Misaelides[26], the author provided a comprehensive analysis of various research efforts involving the use of natural zeolites in soil remediation. Misaelides emphasized that the modification of zeolites through different techniques, such as ion exchange, acid treatment, and surface modification, enhances their ability to remove pollutants from soil. The author further argued that the application of modified zeolites in soil remediation has shown promising results in removing heavy metals, organic pollutants, and various types of contaminants from polluted soils. Therefore, modifying zeolite to treat heavy metal pollution in the environment is extremely important. Modified zeolites refer to zeolite materials that have undergone intentional physical or chemical transformations to alter their properties, enhance their performance, or tailor them for specific applications.
The objective of this work is to present a brief overview of the use of modified zeolite as a remediation technique for heavy metal contamination in soil environments. This work aims to explore different methods of zeolite modification to enhance their properties, allowing effective immobilization, adsorption or reduction of heavy metal contaminants in soil. The scope of the article focuses on: current knowledge related to the application of modified zeolite as a possible solution to overcome heavy metal pollution in soil through adsorption mechanism, advantages and limitations of them through case studies. In addition, the article also highlights factors affecting the adsorption capacity of heavy metals and future research prospects of modified zeolite.
-
Methods of modifying natural zeolites can be categorized into two major groups: modification using organic and inorganic compounds. Firstly, the modification of zeolites using inorganic compounds is executed through: ion exchange; wherein zeolites are converted into their monocation forms (e.g., H+, K+, Cu2+, NH4+, etc.) via treatment with salt or acid solutions. The outcome of this modification alters ion exchange selectivity, molecular sieving, and adsorption properties of zeolites. Treatment with inorganic solutions, in the presence or absence of an oxidizing agent, can also modify the surface properties of zeolites[27,28].
Secondly, the modification of zeolites using organic compounds involves: treatment with simple organic cations (e.g., tetramethylammonium, tetraethylammonium, etc.) to impart hydrophobicity to the zeolite surface[29−31]. Such an adsorbent begins to effectively hold organic compounds. Treatment with more complex organic compounds (e.g., water-soluble polyamines). In this case, the surface of zeolite becomes hydrophobic and zeolites acquire anion exchange properties[31−33]. However, information regarding the extent of preservation of the initial cation exchange characteristics of zeolites is not found in the referenced literature[34].
For more environmentally friendly applications, zeolite was modified using metal cations or metal oxides through straightforward, effective, and relatively cost-efficient processes[35,36]. The charge characteristics of modified zeolites depend on the type of modifying agent and preparation conditions[37].
The techniques for zeolite modification aim to enhance adsorption capacity
-
There exist a multitude of techniques for zeolite modification. Modifications encompass alterations in the chemical surface, structure, or incorporation of supplementary functional groups into the zeolite framework[8, 27−33, 38−40]. These modifications are often tailored to specific requirements of intended applications, aiming to optimize material performance and efficacy. Modified zeolites find applications across diverse fields including environmental remediation, water filtration, gas separation, catalysis, and more. Several common modification methods are presented in Table 1.
Table 1. Some zeolite modification techniques.
Zeolite modification technique Proceed Effective Ion exchange Determined ions are delivered to the zeolite surface through ion exchange processes Enhances the selectivity of the material towards specific ions or molecules Surface functionalization Adding functional groups to the surface Increases the material's affinity for specific pollutants or molecules, making it more effective in adsorption or catalytic reactions Chemical treatment Zeolites are treated with acids, bases or other chemicals to change their surface properties or expand their porous structure. Increase the adsorption capacity or interaction of the material with various substances, including light treatment, silanization, grafting Impregnatio Additional materials are introduced or impregnated onto the zeolite surface Enhances the properties of modified materials or provides additional functions. Nanostructure Create nano-sized particles or structures from zeolite Amplify their surface area, thereby enhancing their performance in various applications Mixture formation Zeolite is combined with different materials, such as polymers or nanoparticles, leading to the development of composite materials with complex properties Improve the adsorption capacity of the material Steam treatment and calcination Steam treatment can change the surface properties of zeolite. The calcination process can remove organic matter and regenerate the zeolite structure. Improve the adsorption capacity of the material. Exemplary studies on the application of modified zeolite for remediation of heavy metal from contaminated soil
-
The application of modified zeolites for pollution treatment in water has been extensively researched and diversified. However, studies on the application of these materials for heavy metal adsorption in soil remain limited. Presented below are some exemplary studies and outcomes regarding the application of modified zeolites for heavy metal remediation in soil.
In a recent study by Ma et al.[41], the authors investigated the potential of modified zeolites for the remediation of heavy metal contamination in soil. This enhancement could be attributed to the introduction of functional groups or nano-particles on the surface of zeolites, thereby augmenting their adsorption capacity. Reeve & Fallowfield devised a remediation scheme for As and Cd contaminated agricultural soils proximate to metal mining and refining activities in China. They employed modified zeolite, specifically hexadecyltrimethylammonium (HDTMA)-modified zeolite, to amend the soil within the mining area and assessed the efficacy of immobilization. Sequential extraction results indicated that the incorporation of HDTMA-modified zeolite not only increased the residual fraction of As (2.7%–5.9%) but also reduced its toxicity-related fraction (2.3%–2.7%) in comparison to unmodified zeolite and blank samples[42]. In 2012, Ioannou et al.[43] employed modified zeolite for the sorption of Cu in soil environments. They crafted altered zeolite systems (I and II) by utilizing a zeolite precursor and goethite. The outcomes demonstrated that the combination of System II with sandy loam soil yielded the most favorable morphological attributes in lettuce with minimal uptake of Cu[43].
In a study by Gao et al.[44], zeolite 4A synthesized from fly ash was modified through a simple ammonia inorganic impregnation method, enabling the incorporation of various types of inorganic amine functional groups into the zeolite structure. This modification enhances the ability of the modified zeolite to remove Hg2+ ions. The modification mechanisms involving impregnation with NH3·H2O, KH792, and NH4Cl on zeolite 4A, as well as the effectiveness in removing Hg2+ ions, were investigated and compared. During the process of soil filtration contaminated with mercury, NH3·H2O-modified zeolite 4A can effectively remove Hg2+ ions and mitigate the risk of additional soil pollution. Li et al.[45] conducted a study on the synthesis of nanoscale zero-valent iron supported by zeolite (Z-NZVI) and its application for simultaneous adsorption of As(III), Cd(II), and Pb(II) from aqueous solutions and soils. These research highlight the significant potential of Z-NZVI for treating water and soils contaminated with multiple heavy metals. Yang et al.[46] implemented a 'waste transformation for soil remediation' strategy by recycling alkali waste red mud and treating heavy metal-contaminated soil. This work primarily converted alkali waste into Fe2O3-ANA for soil treatment, showcasing its value in resource recycling and environmental preservation.
Additionally, experiments conducted by various researchers have aimed to explore the effects of mixing soil with nano-zeolite for the purpose of adsorbing heavy metals in the soil. Most of the results indicate that the nano-material will lead to an increase in the soil's pH efficiency and significantly reduce the concentration of heavy metals in the soil. Notably, this material has no discernible impact on the main flow, which is why nano-zeolite does not participate in the chemical reactions of the soil and water[27, 47−50]. Therefore, future studies might examine the influence of various forms of nano-zeolite and the effective adsorption capabilities of each type on different soil types contaminated with heavy metals.
Dang et al.[51] utilized zeolite and Mg/Al LDH-zeolite to immobilize Cd and Pb ions in artificial soil. The results revealed that the optimal soil pH, adsorbent mass ratio, incubation time, and soil moisture content for immobilizing Cd and Pb ions were 7.0, 3%, 30 d, and 70%, respectively. Precipitation, coprecipitation, and electrostatic attraction were the primary mechanisms of Cd and Pb immobilization on Mg/Al LDH-zeolite, leading to the formation of metal carbonates (CdCO3 and PbCO3). This was attributed to the surface functional groups of the adsorbent and the presence of Fe and Al oxyhydroxides, Mn oxides, as well as Si and O elements in the composition of Mg/Al LDH-zeolite. The Cd and Pb immobilization efficiency with Mg/Al LDH-zeolite was 1.5 to 1.6 times higher than with zeolite. Mg/Al LDH-zeolite exhibited an enhanced capability for immobilizing Cd and Pb ions in a contaminated environment.
Advantages and limitations of current studies
-
In the field of soil remediation, the use of modified zeolite has shown promising results in addressing soil pollution issues. Firstly, studies have demonstrated that modified zeolite exhibits higher adsorption capacity and selectivity for heavy metals compared to natural zeolite. This enhancement could be attributed to the introduction of functional groups or nano-sized particles onto the zeolite surface, thereby enhancing its adsorption properties. Moreover, research has shown significant improvements in the ion exchange capacity, surface area, and number of adsorption sites of modified zeolite. These improvements render it more efficient in sequestering heavy metals within the soil. Additionally, studies have highlighted the stability, recyclability, and cost-effectiveness of modified zeolite, positioning it as a sustainable solution for soil remediation. In conclusion, the findings from these studies contribute valuable insights into the potential of modified zeolite as a promising tool for addressing heavy metal pollution in soil.
However, there are potential challenges that need to be considered for optimizing its application. One such challenge is selecting an appropriate technique for modifying zeolite. According to Usman et al., there are various methods available for zeolite modification, including ion exchange, impregnation, and surface modification. Each technique comes with its own advantages and limitations, and the choice of method must be based on the specific pollutants present in the soil and their chemical characteristics[52]. Furthermore, the effectiveness of modified zeolite in soil remediation is influenced by factors such as pH, temperature, and moisture content. Proper understanding and control of these environmental factors are crucial to ensure the optimal performance of modified zeolite in soil treatment processes. Additionally, the long-term stability and durability of modified zeolite in soil need to be studied. It's essential to assess the filtering capacity of modified zeolite and its potential impact on the surrounding environment. Future research directions should focus on investigating the transport of modified zeolite in soil, as well as its potential effects on soil microorganisms and plant development. By addressing these challenges and exploring future research avenues, the application of modified zeolite for soil treatment could be further enhanced. Furthermore, these studies have mainly concentrated on specific heavy metals. Hence, further research is needed to explore the efficacy of modified zeolite for different soil types and varied environmental conditions, thereby enhancing its practical applicability.
-
The mechanisms of heavy metal adsorption on modified zeolite encompass three primary processes (Fig. 1): precipitation, ion exchange and surface complexation[41, 43, 51, 53−55]. Zeolites exhibit a high capacity for cation exchange, enabling efficient removal of heavy metal ions from solutions. Exchange occurs when cations on the zeolite surface are replaced by heavy metal cations present in the solution. This process is driven by differences in charge and ion size between zeolite cations and heavy metal cations. On the other hand, surface complexation involves the formation of complex compounds between heavy metal ions and functional groups on the zeolite surface. Functional groups such as hydroxyl (-OH) and carboxyl (-COOH) can serve as binding sites for heavy metal ions. These binding sites create stable complexes due to the coordination between functional groups and heavy metal ions, leading to the adsorption of heavy metals on the zeolite surface. This mechanism of heavy metal adsorption on modified zeolite through ion exchange and surface complexation plays a crucial role in the removal of heavy metals from aqueous solutions[53, 56−58]. The effectiveness of these mechanisms for heavy metal removal in soil has also been demonstrated[41, 51, 55, 59].
Figure 1.
Mechanisms for heavy metals by precipitation (I), ion exchange (II) and surface complexation (III)[60].
Factors influencing the process of heavy metal adsorption in contaminated soil by modified zeolite
-
The adsorption of heavy metals onto modified zeolite is influenced by several factors, including pH, temperature, moisture, and contact time[25, 51]. According to Ören & Kaya, pH plays a crucial role in the process of heavy metal adsorption on zeolite[25]. The pH of the solution affects the surface charge of zeolite, thereby influencing its adsorption capacity. For instance, at low pH values, the surface of zeolite becomes positively charged, leading to an increased affinity for heavy metal ions. Conversely, at high pH values, the surface charge becomes negative, reducing the adsorption capacity. Temperature is another significant factor affecting the adsorption of heavy metals. It has been observed that increasing the temperature accelerates the adsorption rate due to the enhanced mobility of metal ions and the higher energy level of the zeolite surface. Additionally, contact time, the duration of interaction between zeolite and the solution, also plays a crucial role in the adsorption efficiency. Longer contact times usually lead to higher adsorption capacity as more metal ions have the opportunity to interact with the zeolite surface. In conclusion, understanding and applying these factors are essential in optimizing the process of heavy metal adsorption onto modified zeolite.
The process of heavy metal adsorption in soil can be influenced by various factors, including the characteristics of the adsorbent material, such as zeolite, and the properties of the heavy metal ions themselves. One significant factor affecting the selective adsorption of heavy metal ions by zeolite is the pore size of the zeolite. Synthesized zeolites with larger pore sizes have been found to exhibit higher adsorption capacities for heavy metal ions[61]. This is because the larger pore size allows hydrated ions to enter the zeolite channels, creating favorable conditions for their adsorption process. Conversely, if the diameter of the hydrated ions exceeds the zeolite's pore size, the metal ions may be excluded from the adsorption process. The dehydration of ions can also enhance their movement into the zeolite channels.
The negative charge and hydration energy of heavy metal ions also play a role in the selective adsorption process by zeolite[62]. It has been observed that heavy metal ions with higher electronegativity, such as Pb2+ and Cu2+, exhibit stronger affinity for the electron clouds of oxygen atoms in the zeolite structure[61]. This is because the electronegative oxygen atoms in the zeolite structure can interact with positively charged heavy metal ions, leading to their selective adsorption. The hydration energy of heavy metal ions also affects their adsorption capacity, with ions possessing higher hydration energy demonstrating greater adsorption ability.
In addition to the characteristics of zeolite, modifying zeolite can also influence the adsorption of heavy metal ions in the soil. For instance, modifying zeolite with calcium or ammonium ions has been found to enhance the adsorption capacity for heavy metal ions[63]. The modified ions promote the ion exchange surface normalization process of zeolite, leading to a higher adsorption capacity for heavy metal ions. The type and concentration of zeolite modifications can also impact the accumulation of heavy metal ions in crops grown in the soil. It has been observed that crops grown on modified zeolite-based substrates exhibit lower transport coefficients for heavy metal ions compared to crops grown on natural zeolite-based substrates.
In summary the adsorption process of heavy metals in soil by modified zeolite is affected by factors such as the pore size of the zeolite, the negative charge and hydration energy of the metal ions and the transformation of the zeolite. Understanding these factors can aid in developing effective strategies for the remediation of heavy metal-contaminated soil.
-
Future prospects in this field are promising, with ongoing research focusing on further enhancing the application of zeolite-based remediation methods. As we continue to deepen our understanding of the mechanisms behind heavy metal adsorption on modified zeolites, we can anticipate the development of more efficient and sustainable techniques for soil remediation. Investigating the interactions between zeolites and various types of heavy metals, as well as their effects on soil microorganisms and plant growth, will be essential for expanding the practical applications of zeolite-based approaches. Moreover, exploring innovative modifications of zeolites and optimizing their performance under different environmental conditions will contribute to the continuous advancement of this field. As we address these challenges and delve into future research directions, the utilization of modified zeolites for soil remediation is likely to see further enhancements and widespread implementation
Modified zeolites have demonstrated significant potential across various industries due to their contribution to environmental sustainability. The utilization of modified zeolites in industries such as catalysis, wastewater treatment, and gas separation can offer substantial environmental benefits[64]. The process of zeolite modification has been shown to reduce the release of harmful elements, making modified zeolites more environmentally friendly[65]. In the future, efforts will continue to develop effective methods for regenerating and reusing modified zeolites, aiding in the reduction of environmental pollution. Concurrently, the use of modified zeolites requires careful assessment to minimize any potential negative impacts on the environment (such as the release of hazardous chemicals during zeolite modification, its long-term stability in different environments, etc.). By addressing these concerns and challenges, modified zeolites have the potential to play a key role in sustainable environmental management.
The development of modified zeolites holds significant potential for diverse applications in various fields. In recent years, researchers have made significant advancements in modifying the structure and properties of zeolites to enhance their catalytic activity and selectivity. According to Khan et al. one of the key challenges in the development of modified zeolites is achieving a high level of control over their structure and composition[66]. This is because even minor changes in the modification process can have a significant impact on their performance. Furthermore, the stability and durability of modified zeolites are crucial factors that need to be addressed to ensure their long-term effectiveness. Additionally, the development of more effective modification methods for large-scale production is essential. Despite these challenges, the prospects for the future of modified zeolites are promising. With further advancements in materials science and nanotechnology, it is anticipated that researchers will overcome these challenges and unlock the full potential of modified zeolites in various applications such as catalysis, gas separation, and environmental treatment.
-
The utilization of modified zeolites presents a substantial potential across diverse industries, offering solutions that contribute to environmental sustainability. The application of modified zeolites in treatment of contaminated soil has demonstrated promising results, showcasing their ability to mitigate environmental pollution and enhance resource efficiency. The optimization of zeolite modification processes, encompassing factors like structure control, stability, and large-scale production, remains a crucial area of research. Overcoming challenges associated with minor alterations impacting performance and ensuring the long-term stability of modified zeolites are paramount for their successful implementation. As scientific progress continues, particularly in materials science and nanotechnology, researchers are poised to address these challenges and harness the full potential of modified zeolites, unlocking innovative solutions for sustainable environmental management.
-
The authors confirm contribution to the paper as follows: reference collection, analysis and review of data: Nguyen TBH; draft manuscript preparation: Van HT. Both authors reviewed and approved the final version of the manuscript.
-
The data used in this study is free from any conflict of interest. The data is available from the corresponding author upon reasonable request.
This work was financially supported by the Vietnam Ministry of Education and Training under project number: B2023-TNA-32.
-
The authors declare that they have no conflict of interest.
- Copyright: © 2024 by the author(s). Published by Maximum Academic Press, Fayetteville, GA. This article is an open access article distributed under Creative Commons Attribution License (CC BY 4.0), visit https://creativecommons.org/licenses/by/4.0/.
-
About this article
Cite this article
Nguyen TBH, Van HT. 2024. Application of modified zeolite in the remediation of heavy metal in contaminated soil: a short review. Technology in Agronomy 4: e002 doi: 10.48130/tia-0023-0021
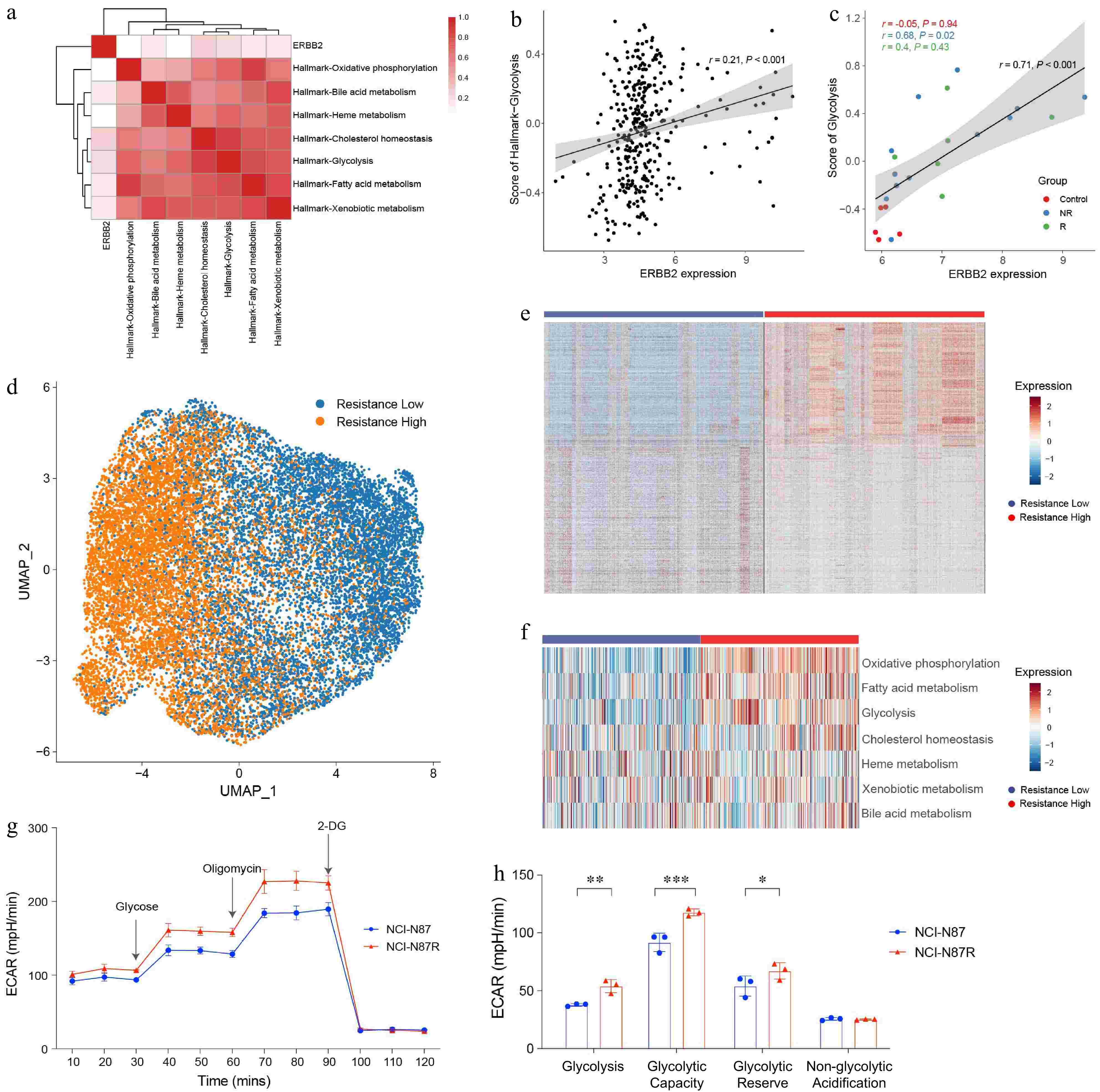