-
Tomato (Solanum lycopersicum L.), is one of the main vegetables found daily in the world's cuisine. It can be eaten both fresh and processed. The heightened demand for this fruit contributes significantly to job creation within the tomato production sector, and it plays a significant role in agribusiness[1]. In addition to its aroma, flavor, and texture, tomatoes are widely accepted for their many benefits for human health. It is a food rich in lycopene, vitamins A and C, and minerals such as potassium, phosphorus, and magnesium, which are important for human nutrition[2].
Climacteric fruits, like tomatoes, are highly perishable. They show a rapid and significant increase in respiration during ripening, with a series of biochemical and visual changes occurring[3]. Post-harvest losses of fruit and vegetables begin at harvest and continue throughout the marketing stage until consumption, i.e. during packaging, transportation, storage and at the consumer level.
Ethylene is the main hormone responsible for ripening and its control is one of the main factors in reducing post-harvest losses. There are several inhibitors used in the conservation of climacteric fruits, which control the action and/or synthesis of ethylene, extending the shelf life of the product[4]. One form of control, for example, is the compound aminoethoxyvinylglycine (AVG).
AVG suppresses ethylene biosynthesis by inhibiting the enzymatic activity responsible for converting S-adenosyl methionine (SAM) to 1-aminocyclopropane-1-carboxylic acid (ACC)[5,6]. Several studies have used AVG to delay ripening and maintain post-harvest quality in climacteric fruits such as bananas[7] and apples[8]. In pears, AVG treatment suppressed the rate of fruit respiration, reduced the loss of firmness, reduced internal browning, senescence disorders, and consequently effectively delayed fruit ripening[9].
Considering the quality and shelf life of the product, there are several damages caused to tomatoes, including handling, transportation, mechanical damage and exposure to high temperatures[3], resulting in rapid ripening with intensification of the red color. As such, tomatoes require a great deal of care and technology for their preservation.
As the effect of AVG can vary depending on the dose and the type of fruit[10], and as there are few studies with AVG in tomatoes, more detailed studies are needed to better understand the issues of the feasibility of using AVG at an appropriate dose. In this context, the aim of this work was to evaluate the quality of tomato fruit cultivar 'Débora' over various postharvest times in refrigerated storage, after immersion in solutions with different concentrations of aminoethoxyvinylglycine (AVG).
-
Tomato fruits (Solanum lycopersicum L. Cultivar 'Débora') obtained from commercial cultivation were used. The fruits were harvested at the salad tomato stage of ripeness, green and ripe, and transferred to the Fruit and Vegetable PostHarvest Laboratory at the Universidade Estadual Paulista 'Júlio de Mesquita Filho', Faculdade de Ciências Agronômicas, Campus de Botucatu, São Paulo, Brazil, where they were selected to standardize the batch, eliminating those with physical damage (dented and/or cracked) and biological damage (diseases and/or pests). The fruits were randomly divided, immersed for 15 min in a solution of hypochlorite with 2% active chlorine, diluted to 7%, washed in running water, and dried in the air, remaining at rest for 24 h to remove the field heat.
Treatment with aminoethoxyvinylglycine (AVG)
-
Each treatment consisted of immersing the fruit for 5 min in a solution of AVG at four concentrations: 0 (control), 500, 1,000, and 1,500 mg·L−1. Each treatment consisted of three replicates. The commercial product used was the plant regulator ReTain®.
After applying the treatments, the fruit was packed in expanded polystyrene trays (two fruits) covered with polyvinyl chloride film (thickness 0.020 mm), stored in a cold room at 15 ± 1 °C and 90% ± 1% RH (average of 31 °C outside) and assessed for quality every 7 d for 28 d.
Weight loss
-
To determine weight loss, a semi-analytical scale (Owa labor model) was used with a maximum load of 2,000 g and a division of 10 mg. The results were calculated as the percentage (%) of weight loss at the start of the experiment and at different intervals during storage using the equation: PM (%) = (Pi ‒ Pj/Pi) × 100, where PP = Weight loss (%); Pi = Initial weight of the fruit (g); Pj = Weight of the fruit in the period following Pi (g)[11].
Respiration rate
-
The respiration rate was determined by quantifying CO2 production in a respirometer, according to a methodology adapted from Bleinroth et al.[12], using the equation: TCO2 = 2.2 × (V0 ‒ V1) × 10/P × T, where, TCO2 = Respiration rate (mL CO2 kg−1·h−1); V0 = Volume spent of HCl for potassium hydroxide titration − standard before CO2 absorption (mL); V1 = Volume spent of HCl for potassium hydroxide titration after CO2 absorption from respiration (mL); P = Fruit weight (kg−1); T = Respiration time (h−1); 2.2 = Inherent CO2 (mL) equivalent (44/2), multiplied by the concentration of hydrochloric acid at 0.1 N; 10 = Adjustment for total potassium hydroxide used in CO2 absorption (mL).
Firmness
-
Firmness was measured at two different points on each fruit and determined using a Texturometer (STEVENS - LFRA Texture Analyzer), with a penetration distance of 20 mm and a speed of 2.0 mm·s−1, using a TA 9/1000 tip. The results were expressed in Newton (N).
Hydrogen potential (pH), Total Soluble Solids (TSS) and Titratable Acidity (TA)
-
The hydrogen potential (pH) of the tomato pulp obtained with the aid of a mixer was determined using a tabletop digital pH meter (model DMPH - 2), according to the methodology of AOAC[11].
The total soluble solids (TSS) content of the extracted tomato pulp was measured using a tabletop digital refractometer (Digital Refractometer DR 202) by direct refractometric reading, according to the methodology of AOAC[11] and the results were expressed in °Brix.
Titratable acidity (TA) was determined by titrimetry. Three milliliters of extracted tomato juice were diluted to 100 mL with distilled water and titrated with 0.1 N sodium hydroxide solution (NaOH) at pH 8.2. The results were expressed as a percentage of citric acid in the fresh pulp, according to the methodology recommended by AOAC[11]. The calculation was made using the formula: AT (%) = [(V × N × meq)/Y] × 100, where V = Volume of sodium hydroxide used in ml, N = Normality of sodium hydroxide and meq = 0.064, Y = Volume of bulk fruit juice mL[11].
External and internal instrumental color
-
The color of the tomato peel and pulp was determined using a Konica Minolta colorimeter (Chroma meter, CR 400) where L*, expressed as a percentage, indicates luminosity values (0% = black and 100% = white), C* is represented by Chroma which defines color intensity. The Hue angle is the value in degrees corresponding to the three-dimensional color diagram and ranges from: 0° to 18° for red-violet, 19° to 54° for red, 55° to 90° for orange, 91° to 126° for yellow, 127° to 162° for yellow-green, 163° to 198° for green, 199° to 234° for blue-green, 235° to 270° for blue, 271° to 306° for blue-violet and 307° to 342° for violet, 343° to 360° red-violet, making 360°[13]. For the approximate reproduction of the color profile, determined by the Konica Minolta colorimeter, the Luminosity, Chroma and Hue angle values of the fruit peel were used to feed into the colorizer.org platform, topic HSL(A), according to the CIELAB diagram.
Experimental design
-
The experimental design was entirely randomized in a 4 × 5 double factorial scheme, with four treatments being the doses of AVG 0 (control), 500, 1,000, and 1,500 mg·L−1 and 5 d of evaluation during the 28 d of refrigerated storage (0, 7, 14, 21, and 28 d) with three repetitions (n = 3).
Statistical analysis
-
The data obtained was subjected to the Shapiro-Wilk normality test using GraphPad Prism software version 8.0.1 (San Diego, CA, USA). With all the data showing normality, it was subjected to analysis of variance (Two-Way ANOVA), and the means were compared using the Tukey test, p < 0.05; and at p < 0.01; using the Sisvar software version 5.6 (Lavras, MG, Brazil). The results were presented as means with standard deviation (mean ± SD). Principal component analysis and correlations was carried out using JMP 10 statistical software (SAS Institute Inc., USA).
-
The results of the analysis of variance with the doses of AVG (0, 500, 1,000, and 1,500 mg·L−1), storage time (days 0, 7, 14, 21, and 28) and the interaction between these factors for the physicochemical parameters assessed at the post-harvest stage of the tomato fruit are presented in Table 1. Except for pH, all the parameters showed significant differences depending on the doses and storage time. There was an interaction between the factors, except for pH and total soluble solids.
Table 1. Results of the analysis of variance (Two-Way ANOVA) of the effect of AVG doses, storage time and the interaction of these factors on the physicochemical parameters evaluated in tomato fruits.
Cause of variation D.F. Weight loss Respiratory rate Firmness Total soluble solids pH Tritrable acidity Dose 3 0.000** 0.000** 0.027* 0.042* 0.778ns 0.046* Time (d) 4 0.000** 0.000** 0.000** 0.049* 0.579ns 0.000** Dose × time 12 0.004** 0.000** 0.000** 0.219ns 0.988ns 0.002** Residue 40 0.150 3.264 0.033 0.122 0.069 0.000 ns represents not significant; * represents p ≤ 0.05 and ** represents p ≤ 0.01. There was an increase in weight loss in tomato fruit at all doses during storage (Fig. 1a). The control fruit (0 mg·L−1) showed less weight loss compared to the fruit treated with AVG, and on day 28 the 1,000 and 1,500 mg·L−1 doses showed greater weight loss compared to the other doses. In a similar previous study, the weight loss of 'Grando F1' tomatoes increased with longer storage time and reached 1.38% in the AVG-treated fruit and 1.21% in the control fruit after 20 d at 12 °C[14], so there seems to be no consistent effect of AVG treatments on weight loss. Different results were obtained in apple cultivar 'Eva', where the doses of 500 and 1,500 mg·L−1 were responsible for the lowest weight loss from the 21st and 28th d[15]. Although positive effects have been observed in some fruit varieties, opposite results have been obtained in other fruits[10].
Figure 1.
(a) Weight loss, (b) respiration rate and (c) firmness of tomato fruit treated with doses of aminoethoxyvinylglycine (AVG) and stored (15 ± 1 °C and RH 90% ± 5 %) for 28 d. Lowercase letters differ the doses in a single storage time and capital letters differ the storage times in a single dose, according to the Tukey test (p < 0.05).
Weight loss is a process that occurs due to the loss of water, stimulated by the process of respiration and transpiration due to the vapor pressure deficit on the surface of the fruit[16]. The loss of water leads to softening of the tissues, affecting the texture, color, and flavor of the fruit, reducing its time on the market. As reported by Taiz et al.[17], AVG also acts to inhibit enzymes that use pyridoxal phosphate as an enzyme cofactor and when applied in high doses, this may have occurred in this study by increasing weight loss, inhibiting various aspects of the plant's metabolism, especially interfering with the rind tissue.
There was an increase in the respiration rate of the fruit on the 7th day of evaluation, with a gradual increase over time until the maximum climacteric peak of 55.31 mL CO2 kg−1·h−1 on the 14th day for the control fruit, followed by a decline on the other days (Fig. 1b). From the 7th to the 28th day, the control had a significantly higher respiration rate, 55% to 64% higher on the 28th day compared to the AVG-treated fruit, showing a positive effect of this compound. The respiration rate can be reduced using various technologies that reduce the metabolic activity of the fruit, such as refrigerated storage and inhibitors of ethylene action[6], like AVG. The continuation of the metabolic process gradually alters the composition of the product, leading to senescence, which was delayed with AVG in this study.
Over time, there was a decrease in the firmness of the fruit from day 14 onwards, which was gradual until day 28 (Fig. 1c). AVG provided greater fruit firmness compared to the control at a dose of 1,500 mg·L−1 on day 7 and 1,000 mg·L−1 on day 21, and on the other days there was no difference between the doses. The difference in firmness between treated and untreated fruit disappears over time[18], which probably occurred on day 28. When applied as a postharvest dip treatment, AVG reduced the rate of fruit softening in 'Grando F1' tomatoes[14], 'Huangguan' pears[9] and sweet orange Citrus sinensis (L.)[19] during storage or shelf life, as a consequence of ethylene inhibition.
The total soluble solids (TSS) content of the control fruit (0 mg·L−1) decreased over time, and for the other doses there was stability, with no significant difference (Fig. 2a). On day 28, the 1,000 mg·L−1 dose provided a 1.0 °Brix increase in SS content compared to the control. The SS content is used as an indirect estimate of sugars, as well as other compounds that are present in the vascular sap, such as vitamins, phenolics and pectin's. Like this study[20], working with 'Tommy Atkins' mangoes at room temperature, observed stability in the SS content when treated with AVG during the 12 d of storage. However, this was probably due to the ripening process of the fruit with the conversion of starch to sugar.
Figure 2.
(a) Total soluble solids, (b) pH and (c) titratable acidity of tomato fruit treated with doses of aminoethoxyvinylglycine and stored (15 ± 1 °C and RH 90% ± 5 %) for 28 d. Lowercase letters differ the doses in a single storage time and capital letters differ the storage times in a single dose, according to the Tukey test (p < 0.05).
The pH showed no statistical difference between the treatments (Fig. 2b). A previous study,[15] also found no significant difference for the doses of AVG, but found a significant effect for the storage time of the 'Eva' apple cultivar. The author observed higher pH values on the 0th and 7th day of storage, with a decrease in value from the 14th day onwards. This reported decrease was linked to the release of organic acids resulting from the degradation of the cell wall, a fact that did not occur in this study under the conditions in which the experiment was carried out.
Titratable acidity (TA) remained stable in the fruit treated with the 1,500 mg·L−1 dose during storage; on the other hand, there was a reduction in the fruit treated with the other doses (Fig. 2c). In the fruit treated with the 1,500 mg·L−1 dose on day 28, the acidity was 0.08% and 0.05% higher, respectively, compared to the two lower doses, 0 and 500 mg·L−1. Corroborating this work, dos Santos et al.[20], working with 'Kent' mango, reported that the 300 mg·g−1 concentration of AVG resulted in fruit with a higher acidity content. Titratable acidity analysis is used to quantify acidity through a predominant acid according to the plant material, determining the percentage of organic acids.
Corroborating the present study, it was previously reported that TA decreased over time and no significant differences were found in the pH of the pulp of 'Grando F1' tomatoes between fruit treated and untreated with 1,000 mg·L−1 AVG at −30 kPa during storage at 12 °C for 20 d and a further 2 d at 20 °C[14]. However, unlike the present study, these authors found no significant differences in SS and TA between fruit treated and not treated with AVG, reporting that changes in organic acids and sugars in tomatoes were not controlled by ethylene[21], a positive effect that certainly occurred in the present study due to the inhibition of ethylene by AVG at a dose of 1,500 mg·L−1, as it kept TA and SS stable.
The results of the Two-Way ANOVA with the doses of AVG (0, 500, 1,000, and 1,500 mg·L−1), storage time (days 0, 7, 14, 21, and 28) and the interaction between these factors for the external and internal color parameters, assessed at post-harvest of the tomato fruit, are shown in Table 2. Except for the effect of the doses and the interaction between the factors for internal luminosity (pulp), all the other parameters showed significant differences. Storage time was significant for all parameters.
Table 2. Results of the analysis of variance (Two-Way ANOVA) of the effect of AVG doses, storage time and the interaction of these factors on the external and internal color parameters evaluated in tomato fruits.
Cause of variation D.F. L peel C peel h° peel L pulp C pulp h° pulp Dose 3 0.001** 0.000** 0.000** 0.866ns 0.030* 0.045 * Time (d) 4 0.000** 0.000** 0.000** 0.000** 0.000** 0.000** Dose × time 12 0.038* 0.010* 0.000** 0.695ns 0.014* 0.000** Residue 40 1.780 4.402 3.294 7.097 2.507 12.640 ns represents not significant; * represents p ≤ 0.05 and ** represents p ≤ 0.01. As for peel color, external luminosity decreased over time (Fig. 3a), and on day 28, the 500 mg·L−1 dose provided significantly lower luminosity compared to the 0 mg·L−1 dose, indicating lower brightness of the fruit periderm (Fig. 3b), but both did not differ from the other doses. There was an increase in external chroma values over time, and on day 28 the AVG doses showed lower chroma compared to the control. Corroborating the present study, in a previous study with 'Grando F1' tomatoes treated with 1,000 mg·L−1 of AVG at −30 kPa during storage at 12 °C for 20 d and a further 2 d at 20 °C after refrigerated storage[14], it was reported that AVG reduced the rate of ethylene production and delayed ripening changes in peel color (L*, C* and h° values, chlorophyll and lycopene content). Therefore, C* values increased while h° and L* values decreased at a lower rate in AVG-treated fruit than in control fruit during storage and shelf life.
Figure 3.
External instrumental color (peel), (a) luminosity, (b) chroma and (c) hue angle of tomato fruits treated with doses of AVG and stored (15 ± 1 °C and RH 90% ± 5 %) for 28 d. Lowercase letters differ the doses in a single storage time and capital letters differ the storage times in a single dose, according to the Tukey test (p < 0.05).
The external °hue decreased until day 14 and remained stable for the rest of the time (Fig. 3c). This behavior leads to a change from green to red, which can possibly be attributed to the degradation of chlorophyll and the biosynthesis of lycopene, responsible for the tomato's red color. On day 14, the doses of AVG showed lower °hue compared to the control, and on day 21 there was no difference between the doses, and on day 28 the highest doses (1,000 and 1,500 mg·L−1) provided color maintenance compared to the control (Fig. 4), indicating that AVG reduced fruit ripening.
Figure 4.
Approximate reproduction of the color profile of the peel of tomato fruit treated with doses of AVG and stored (15 ± 1 °C and RH 90% ± 5%) for 28 d, determined using a Konica Minolta colorimeter, with Luminosity, Chroma and °Hue values fed into the colorizer.org platform.
For internal color, there was no statistical difference between AVG doses only for brightness, showing that there was no negative effect of AVG on fruit brightness. In relation to time, there was a decrease over the days, indicating a loss in the internal brightness of the fruit (Fig. 5a). On the 28th day, the internal chroma was higher in the 0 mg·L−1 control and the doses did not differ (Fig. 5b). There was an increase in color intensity on days 14 and 21. The internal ºhue gradually decreased until day 14 and remained stable on the other days (Fig. 5c), and there was no significant difference between the doses on days 14, 21 and 28. Aglar[22] reported that spraying 225 mg·L−1 of AVG on 'Li' jujube trees pre-harvest and keeping the fruit in cold storage for 45 d at 0 ± 0.5 °C and 90% ± 5% relative humidity (RH) reduced the development of fruit color.
Figure 5.
Internal instrumental color (pulp), (a) luminosity, (b) chroma and (c) hue angle of tomato fruit treated with doses of AVG and stored (15 ± 1 °C and 90% ± 5% RH) for 28 d. Lowercase letters differ the doses in a single storage time and capital letters differ the storage times in a single dose, according to the Tukey test (p < 0.05).
Principal component analysis (PCA) of 12 parameters evaluated in tomato fruit treated with doses of AVG (0, 500, 1,000, and 1,500 mg·L−1) and stored for 28 d (day 0, 7, 14, 21, and 28), allowed the general observation of the data in a smaller dimension, separated by treatment in different quadrants (Fig. 6). The variability was explained by two principal components (PC) with eigenvalues > 1.0. These two significant PCs (PC1 and PC2) accumulated 76.9% of the total variation.
Figure 6.
(a) Score plot and (b) load plot of the principal component analysis of 12 parameters evaluated in tomato fruit treated with doses of AVG and stored (15 ± 1 °C and RH 90% ± 5%) for 28 d. Score graph (a): 0 mg·L−1 dose on days 0 (A), 7 (E), 14 (B), 21 (C) and 28 (D); 500 mg·L−1 dose on days 0 (P), 7 (T), 14 (Q), 21 (R) and 28 (S); 1,000 mg·L−1 dose on days 0 (F), 7 (J), 14 (G), 21 (H) and 28 (I); and 1,500 mg·L−1 dose on days 0 (K), 7 (O), 14 (L), 21 (M) and 28 (N). Load graph (b): weight loss (WL), respiratory rate (Resp), firmness (Firm), total soluble solids (SS), hydrogen potential (pH), titrable acidity (TA), lightness external (L peel), chroma external (C peel), hue angle external (°h peel), lightness internal (L pulp), chroma internal (C pulp) and hue angle internal (°h pulp).
PC1 was responsible for 61.6% of the total variation and was effective in separating the treatments on days 0 and 7 into positive scores and the treatments on the remaining days (14, 21, and 28) into negative scores (Fig. 6a). Analysis of the PC1 loadings (Fig. 6b) suggests that this separation is mainly due to the analyses of firmness, external and internal luminosity and external and internal °h which have strong positive loadings (> 0.80) and the analyses of external and internal chroma with strong negative loadings in the PCA (< 0.75). The PC1 scores and loadings showed a greater effect for time than for AVG doses.
PC2 in the score graph (Fig. 6a) was important mainly for separating dose 0 on day 28 (D), since this treatment had a positive score, from doses 1,000 and 1,500 on day 28 (I, N) which had the strongest negative scores, corroborating the respiration rate mean test (Fig. 1b). PC2 represented 15.3% of the total variation and was mainly related to positively charged respiration rate and negatively charged weight loss (Fig. 6b). Therefore, principal component analysis was effective in confirming the results presented here.
By grouping the dependent variables via correlation, PCA indicated that there were positive correlations between weight loss, external and internal chroma, which correlated negatively with firmness, external and internal luminosity, external and internal hue angle, total soluble solids, and titratable acidity, and all the latter correlated positively with each other (Supplemental Fig. S1). This infers that, as weight loss increased, firmness decreased, an effect that was minimized with AVG, which may be related to the inhibition of the fruit's ethylene production rate and the expression of genes associated with chlorogenic acid metabolism, perception and signal transduction and membrane breakdown in the central tissue, as well as a decrease in malondialdehyde activity and polyphenol oxidase enzyme activity[9].
-
Under the conditions in which the experiment was carried out, it can be concluded that immersing 'Débora' tomato fruit in AVG solution at a dose of 1,500 mg·L−1 delayed ripening, reduced the fruit's respiration rate, and the changes in external and internal chroma, and it did not reduce weight loss. The doses of AVG did not negatively affect the luminosity and pH of the fruit pulp. The untreated fruit became soft and ripe red 7 d earlier than the treated fruit during the 28 d of storage at 15 ± 1 °C and 90% ± 1% RH. The feasibility of using AVG to market tomato fruit to distant markets or to local or retail markets for longer was demonstrated. More studies are needed on the use of AVG in different conditions and with other tomato cultivars.
-
The authors confirm contribution to the paper as follows: study conception and design: Aparecida dos Santos J, Rocha Lacerda V; methodology: Rocha Lacerda V, Aparecida dos Santos J, Gaona Acevedo AF, Lopes Vieites R; software: Rocha Lacerda V; validation: Rocha Lacerda V, Aparecida dos Santos J, Sílvia Angélica de Oliveira H, Gaona Acevedo AF, Rocha Lacerda V; formal analysis: Rocha Lacerda V, Aparecida dos Santos J, Gaona Acevedo AF; investigation: Rocha Lacerda V, Aparecida dos Santos J, Sílvia Angélica de Oliveira H; resources: Rocha Lacerda V; data curation: Rocha Lacerda V, Aparecida dos Santos J; draft manuscript preparation: Rocha Lacerda V, Aparecida dos Santos J, Sílvia Angélica de Oliveira H; final manuscript preparation: Rocha Lacerda V, Sílvia Angélica de Oliveira H, Lopes Vieites R; visualization: Rocha Lacerda V, Aparecida dos Santos J, Sílvia Angélica de Oliveira H, Gaona Acevedo AF; supervision: Lopes Vieites R; project administration: Aparecida dos Santos J; funding acquisition: Lopes Vieites R. All authors reviewed the results and approved the final version of the manuscript.
-
All data generated or analyzed during this study are included in this published article, and are available from the corresponding author on reasonable request.
This study was financed in part by the Coordenação de Aperfeiçoamento de Pessoal de Nível Superior - Brazil (CAPES) - Financing Code 001. To the Conselho Nacional de Desenvolvimento Científico e Tecnológico – CNPq, for the financial support for this work.
-
The authors declare that they have no conflict of interest.
- Supplemental Fig. S1 Correlations by color map cluster of twelve parameters evaluated in tomato fruit treated with doses of AVG and stored (15 ± 1 °C and RH 90 ± 5 %) for 28 days.
- Copyright: © 2024 by the author(s). Published by Maximum Academic Press, Fayetteville, GA. This article is an open access article distributed under Creative Commons Attribution License (CC BY 4.0), visit https://creativecommons.org/licenses/by/4.0/.
-
About this article
Cite this article
Rocha Lacerda V, Aparecida dos Santos J, Sílvia Angélica de Oliveira H, Gaona Acevedo AF, Lopes Vieites R. 2024. Doses of aminoethoxyvinylglycine (AVG) in tomato postharvest storage. Technology in Horticulture 4: e011 doi: 10.48130/tihort-0024-0008
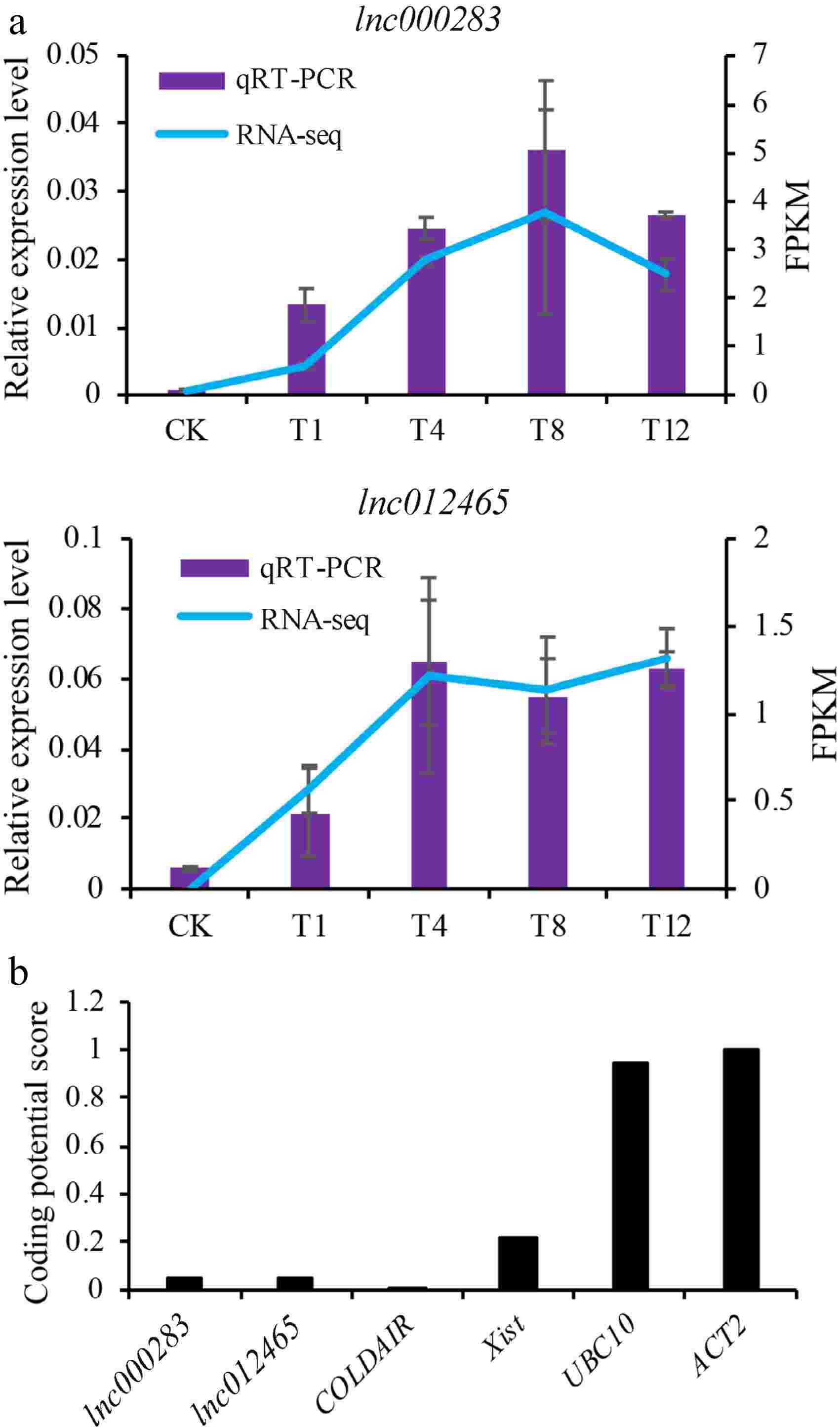