-
Rice (Oryza sativa) is grown in many African countries with substantial increased productivity recently[1], but the overall rice demand in Africa has outstripped local production, requiring 40% imported rice. Tanzania is the most important rice production zone in East Africa; rice is the second most cultivated food and commercial crop in Tanzania after maize. However, rice productivity in Tanzania is still low, with the average yield in the range of 1.5−2.8 t·ha−1, due in large part to the fact that small-scale and low-technology farmers account for ca. 70% of the rice growing area. On these farms, fertilisers are often not used because of the cost, limiting yields. Rice blast caused by Magnaporthe oryzae is another challenge facing rice farmers[2−4]. Good blast control may be achieved with intensive fungicide programmes, but this is both expensive, particularly for smallholder farms, and undesirable[5].
The use of beneficial microbes is being promoted for their potential in sustainable agriculture, by exploiting their biocontrol effects against specific diseases and/or plant growth promoting effects. In addition to conventional applications of biopesticides to soils (e.g., drenching) and plants (e.g., dipping, spraying), coating seeds with low amounts of beneficial microbes can be an efficient way to delivering beneficial microbes for improving seed germination, seedling establishment and suppression of root diseases[6, 7]. Treating Brassica napus seeds with biocontrol organisms and fungicides were, however, less effective than soil drench in reducing clubroot caused by Plasmodiophora brassicae[8]. Bio-priming rape seed with Serratia plymuthica led to reduced development of Verticillium dahliae[9]. Coating eggplant seeds with Paenibacillus alvei or a nonpathogenic Fusarium oxysporum strain led to significant suppression of Verticillium wilt[10].
To control rice blast, biopesticides may be applied as a seed priming treatment, dipping treatment at transplanting, and foliar sprays post-transplanting[11−14]. Promising organisms against rice blast include strains from several common sources for biocontrol agents: Bacillus, Trichoderma, Streptomyces and Pseudomonas[12, 13, 15, 16]. Several rhizobacteria showed a good efficacy against rice leaf blast when applied as soil drench or foliar spray but the efficacy varying with application methods[17]. A recent pilot study in Kenya showed that dipping roots of rice seedlings in commercial formulated B. subtilis or T. asperellum products led to reduced blast development and increased yield. Following this pilot study, we conducted further field studies and showed that applying formulated B. subtills or T. asperellum commercial products as transplanting dipping or as post-transplanting foliar sprays led to large increases in rice yield only at subsistence farming low-yield sites (Tanzania) and the benefit of using the same organism product as dipping or foliar spray varied greatly with seasons[18]. Reduction in blast development due to biocontrol was achieved but did not necessarily lead to corresponding increases in yield[14]. It is often recommended to combine biopesticides to achieve better performance, exploiting potential synergies among different microbial organisms. However, such a laudable objective of exploiting synergies has rarely been achieved[19]. Mixed use of formulated B. subtills or T. asperellum products did not lead to additional benefits in terms of blast control or rice yield[18].
The present study aims to develop strategies of applying commercial biopesticides for rice growers in Africa, extending our previous research[18] in the following three specific aspects on the use of formulated microbial products in rice production. Firstly, alternate use of formulated B. subtills and T. asperellum products as transplanting dipping or post-transplanting foliar spray were assessed for potential synergistic effects in reducing blast and increasing yield. In the previous research[18], the same biopesticide product was used in dipping and post-transplanting foliar spray. Secondly, we reduced the number of post-transplanting foliar sprays from the previous five/six sprays to two sprays. Finally, the effect of coating rice seeds with one S. nematodiphila strain on blast development and rice yield was compared with using microbial products as transplanting dipping and post-transplanting foliar spray. In our previous study, newly formulated product of this S. nematodiphila strain performed similarly to formulated B. subtills and T. asperellum products when applied as dipping or post-transplanting foliar spraying.
-
Experiments were conducted at one site in Kenya for two cropping seasons and three sites in Tanzania for one crop season in 2022, at the same sites as the experiments reported previously[18]. A randomised block design was used for all sites. Within each block, there was one plot (5 m × 5 m) randomly assigned to one of the treatments; planting space was 20 cm × 15 cm. There was a gap of 75 cm between neighbouring plots.
In Kenya, the study objective was to compare a seed-coating treatment with the formulated product of one specific Real IPM [The Real IPM Co. (Kenya) Ltd., Thika, Kenya] S. nematodiphila strain (code SN01) with three other Real IPM formulated products of specific B. subtilis strain Bs01 (trade name: REGAIN®), T. asperellum strain T-900 (trade name: Sustain®), and the same S. nematodiphila strain SN01 when applied as dipping at transplanting and/or post-transplanting foliar spray. In Tanzania, the main objective was to assess whether there was additional benefit in the alternate use of formulated B. subtilis and T. asperellum products as transplanting dipping or post-transplanting foliar spray. All formulated B. subtilis, T. asperellum and S. nematodiphila products contained 1.0 × 1010 CFUs ml−1 of specific microbes. The formulated S. nematodiphila product and seed coating product with the S. nematodiphila strain were not included in Tanzania as they were not approved for use there.
For transplanting dipping treatments, seedling roots were dipped in an appropriate product (5 ml formulated product in 1 L water) for 30 min before transplanting. For post-transplanting foliar application, unlike in the previous study[18], microbial products or fungicides were applied only twice with a knapsack sprayer to protect panicles when they were emerging from the boot, about nine weeks after transplanting; two sprays were applied 7 d apart. Each plot received approximately 7 L each time at the same concentration as dipping treatments.
Kenya
-
A single cultivar (cv. Basmati 370) was used at the National Irrigation Board site (longitude −37°37'2.53" E and latitude −0°49'24.23" S). In this region, the main agricultural activity is monocropping of rice grown in paddies that are irrigated for six months. The soil at the site is of the black cotton soil type (vertisol).
There were five blocks and nine treatments. There were three formulated products (B. subtilis, T. asperellum and S. nematodiphila), each applied as (i) transplanting dipping only, and (ii) both as transplanting dipping and post-transplanting foliar spray. There were three additional treatments: (i) seed coating with S. nematodiphila only, (ii) water control [dipping in and foliar spray with water], and (iii) fungicide control [post-transplanting spray only − 'Absolute', a mixture of azoxystrobin (200 g·L−1), difenoconazole (125 g·L−1) and hexaconazole (50 g·L−1)]. For the first cropping season, seeds were sown in early February 2022 and transplanted in early March 2022. For the second cropping season, seeds were sown in late August 2022 and transplanted in late September 2022. Fertilisers were applied via broadcasting to the trial on three occasions: muriate of potash at 25 kg per acre, three days after transplanting, and sulphate of ammonia at 50 kg per acre, two and seven weeks after transplanting.
Number of tillers and the height of the highest tiller for each plant was assessed weekly immediately from transplanting, giving a total nine assessments. In each plot, the same five plants (randomly selected on the first assessment date) were assessed for tiller development at all nine time points. For each plot, time to the first and median flowering was recorded. Rice blast assessment commenced four weeks after transplanting and thereafter weekly on five leaves, one leaf from each of five plants randomly selected on each assessment occasion for each plot. As blast was not severe, only presence of rice blast was recorded. In total there were five blast assessments. For each plot, 10 panicles (heads) were randomly selected, one from each of 10 randomly selected plants, to estimate number of grains per panicle. The 1000 seed weight and unshelled (raw) gross grain yield were obtained for each plot.
Tanzania
-
Experiments were conducted at three sites [Babati (−3.8541° S, 35.5235° E), Kikwe (3.3711° S, 36.8285° E) and Moshi (3.4197° S, 37.3676° E)]; the soil at all three sites is of the black cotton soil type (vertisol). Seeds were sown on 1/12/2021 (Kikwe), 21/12/2021 (Moshi), and 11/01/2022 (Babati). A single cultivar [cv. SARO 5 (TXD 306)] was used at all three sites.
At each site, there were three blocks and nine treatments. There were two formulated products (B. subtilis and T. asperellum) that were applied individually in two treatments: only transplanting dipping, and both transplanting dipping and post-transplanting foliar spray. There were a further two treatments with alternate use of the two products: B. subtilis dipping/T. asperellum spray, and T. asperellum dipping/B. subtilis spray. Finally, there were three control treatments: two water controls and one fungicide control (SC250ECORE 250 g·L−1 (difenoconazole), applied at 0.5 L·ha−1). One of the two water controls was initially reserved for the S. nematodiphila seed coating treatment; unfortunately, this product was not registered in Tanzania in time for this experiment. Urea was applied through broadcasting as a top dressing six weeks after transplanting; lambda cyhalothrin or deltamethrin was applied to control stalk borers four weeks after transplanting.
Number of tillers and the height of the highest tiller for each of five plants were assessed three times; five plants were randomly selected for tiller assessment at each time point. At Kikwe, they were measured 6, 14 and 19 weeks after transplanting; at Moshi, 6, 10 and 15 weeks after; and at Barbati, 4, 18 and 28 weeks after. For each plot, times to 30% flowering and to maturity were recorded. Number of seeds per head and percentage of grains with rice blast symptoms were recorded at harvest: five panicles were selected randomly (one from a single plant) for each plot to count number of grains per panicle and with blast symptoms. Finally, both unshelled (raw) and shelled (net) grain yields were obtained for each plot.
Data analysis
-
For all experiments, preliminary repeated measurement analysis of variance (ANOVA) of tiller number and height showed that there were no significant interactions between assessment dates and treatments. Thus, only the tiller number and height on the final assessment date were statistically analysed and presented. All statistical analyses and graphing were conducted in R[20].
Kenya
-
The data were subject to two stages of ANOVA. For yield, number of seeds per panicle, and days to flowering or maturity, log transformation was applied to ensure that residual errors follow (or closely follow) normal distributions. A logit transformation was applied to the incidence of leaves with blast symptoms before ANOVA. No transformation was needed for the 1000 seed weight, tiller number and height. Firstly, ANOVA was applied to all the treatment data pooled over the two crop seasons where cropping season was treated as a blocking factor in addition to the within-experiment block and treatment factors. When ANOVA indicated significant differences among the nine treatments, the Tukey HSD (honestly significant difference) test was then applied to conduct pairwise comparisons. Secondly, ANOVA was applied to the six treatments involving three microbial products applied as dipping or as foliar spray. In this analysis the six microbial treatments were considered as a two factorial design [three products, and two application strategies (dipping only, dipping + foliar spray) to assess whether three products differed overall in their effects and whether there were interactions between the three products and two application strategies.
Tanzania
-
Similarly a two-stage ANOVA analysis as for the Kenya data were applied. In addition to unshelled (gross) and shelled (net) grain yield, the ratio between the net and gross grain weight was also calculated and analysed. For net gross ratio, log transformation was applied to ensure that residual errors follow (or closely follow) normal distributions. For all other variables, transformation was the same as for the Kenya data. In the first analysis of all nine treatments, the site was treated as a blocking factor in addition to the within-site block and treatment factors. The second ANOVA was applied to the six microbial treatments to determine the effects of multiple treatments and possible synergy from alternate use of Bacillus and Trichoderma products. In this ANOVA, in addition to site and within-site block factors, there were two treatment factors: products used as (i) dipping (Bacillus or Trichoderma), and (ii) as foliar spray (Water, Bacillus or Trichoderma). The main effect of the foliar spray (two degree-of-freedoms, DF) was further decomposed into two single DF orthogonal comparisons: (i) water vs the two microbial products, and (ii) Bacillus vs Trichoderma. The interaction of the dipping factor with the single DF Bacillus vs Trichoderma foliar spray comparison indicates whether there were synergies in alternative us of Bacillus and Trichoderma products over time.
-
Both tiller number and height increased nearly linearly with time and for both variables there were no significant interactions between treatment and time. The overall average final number of tillers per plant across all treatments was 36.7 (± 0.50). The final tiller number differed (p < 0.001) among the nine treatments: all the six treatments with microbial dipping and/or foliar spray led to more tillers than the water control (Table 1). Overall, there were no additional benefits of applying foliar spray in addition to dipping. The three microbial products did not differ from each other irrespective of the application strategy. In contrast, tiller height did not differ among the nine treatments and among the three products; the overall average final tiller height was 116.0 cm (± 1.80).
Table 1. Significant pairwise comparisons among the nine treatments applied to rice plants at one site in Kenya across two cropping seasons in 2022, based on the Tukey HSD test. The nine treatments included three biopesticides (Bacillus subtilis, Trichoderma asperellum and Serratia nematodiphila), each applied as dipping at transplanting, and post-transplanting foliar spray as well as dipping, and two controls (water and foliar fungicide spray). The final treatment was seed coating with S. nematodiphila.
Treatment 1
(dipping : spraying)Treatment 2
(dipping : spraying)Differences p value Tiller number on the final assessment date Water control Bacillus : Bacillus −0.104 0.0247 Water control Bacillus : Water −0.111 0.0124 Water control Serratia : Serratia −0.099 0.0383 Water control Serratia : Water −0.114 0.0094 Water control Trichoderma : Trichoderma −0.107 0.0189 Water control Trichoderma : Water −0.109 0.0146 Number of seeds per panicle (on the natural logarithm scale) Trichoderma : Water Serratia : Water 0.156 0.0320 Water control Bacillus : Bacillus −0.200 0.0015 Water control Bacillus : Water −0.181 0.0062 Water control Serratia : Serratia −0.184 0.0049 Water control Serratia : Water −0.188 0.0036 Water control Trichoderma : Trichoderma −0.217 0.0004 Water control Trichoderma : Water −0.241 0.0001 Water control Fungicide −0.155 0.0331 Incidence of tillers with blast symptoms (on the logit scale) Water control Bacillus : Bacillus 0.685 0.0000 Water control Bacillus : Trichoderma 0.785 0.0000 Water control Serratia coating 0.554 0.0000 Water control Serratia : Serratia 0.618 0.0000 Water control Serratia : Water 0.608 0.0000 Water control Trichoderma : Trichoderma 0.639 0.0000 Water control Trichoderma : Water 0.532 0.0000 Water control Fungicide 0.597 0.0000 Days to first flowering
-
On average, it took 79.0 d (± 0.53) to reach flowering onset; the length of this time did not differ significantly among the nine treatments as well as among the three products.
Yield data
-
Overall, there were 89.1 seeds (± 1.91) per panicle. There were significant (p < 0.001) differences among the nine treatments in the number of seeds per panicle, due primarily to the fact that the water control had fewer seeds than all the other treatments, except the seed-coating (Fig. 1; Table 1). There were no additional benefits of applying foliar spray in addition to dipping. There were no significant differences among the three products irrespective of the application strategy. The grand average 1000 seed weight was 20.1 g (± 0.21) and there were no significant differences among the three products or effects of additional foliar applications.
Figure 1.
Number of grains per head of rice plants, treated by one of the four biopesticides at different times (seed coating, dipping at transplanting, post-transplanting foliar spray, or both dipping and spray) at one site in Kenya over two cropping seasons in 2022. Two controls (water as negative, and fungicide foliar spray as positive) were included for comparison. The bar represents one standard error, and P value is associated with the F-test in ANOVA, indicating the overall differences among the nine treatments.
The average gross grain yield was 16.1 kg (± 0.39) per plot; there were no significant differences among the nine individual treatments (Fig. 2). However, ANOVA of combined data over all three microbes (including seed-coating) showed that applying beneficial microbes once (seeding coating or dipping) or twice (dipping and foliar spray) led to a significant increase (p < 0.05) in the gross yield over the water control. The average gross yield per plot was 13.9 kg (± 0.78), 16.6 kg (± 0.61) and 16.4 kg (± 0.57) for the water control, dipping or seed-coating, and both dipping and spray treatments, respectively. No additional benefit was obtained when foliar spray was applied in addition to transplanting dipping. There were no significant differences among the three products when applied as dipping or foliar spray.
Figure 2.
Gross yield (kg per plot) of rice plants, receiving one of the four biopesticides applied at different times (seed coating, dipping at transplanting, post-transplanting foliar spray, or both dipping and spray) at one site in Kenya over two cropping seasons in 2022. Two controls (water as negative, and fungicide as positive) were included for comparison. The bar represents one standard error and P value is associated with the F-test in ANOVA, indicating the overall differences among the nine treatments.
Blast development
-
Across all treatments, about 7.9% of leaves had blast lesions. There were significant (p < 0.001) differences among the nine treatments in the blast incidence – the water control had a higher blast incidence than all the other treatments (Fig. 3, Table 1). Seed-coating or dipping alone managed rice blast as satisfactorily as the fungicide treatment; there were no additional reductions in blast with additional foliar application. The three microbial products did not differ in their effects on blast development when applied as dipping or foliar spray.
Figure 3.
Percentage of tillers with blast symptoms for those rice plants receiving one of the four biopesticides applied at different times (seed coating, dipping at transplanting, post-transplanting foliar spray, or both dipping and spray) at one site in Kenya over two cropping seasons in 2022. Two controls (water as negative, and fungicide as positive) were included for comparison. The bar represents one standard error, and P value is associated with the F-test in ANOVA, indicating the overall differences among the nine treatments.
Tanzania experiments
Tiller number and height
-
For both tiller number and height there were no significant interactions between treatment and time. The average final number of tillers per plant was 18.8 (± 0.62). The final tiller number differed (P < 0.001) among the eight treatments: all four treatments with both transplant dipping and foliar applications of Bacillus or Trichoderma led to more tillers than the water control (Table 2). Foliar spray in addition to dipping led to further increases (p < 0.05) in the number of tillers; but there were no synergies in alternate use of Bacillus and Trichoderma.
Table 2. Significant pairwise comparisons for all eight treatments applied to rice plants at three trial sites in Tanzania in 2022, based on the Tukey HSD test. The eight treatments included six microbial treatments and two controls (water and fungicide foliar spray).
Treatment 1
(dipping : spraying)Treatment 2
(dipping : spraying)Differences p value Number of tillers (on a log scale) Bacillus : Bacillus Water control 0.307 0.0108 Bacillus : Trichoderma Water control 0.328 0.0050 Trichoderma : Bacillus Water control 0.403 0.0002 Trichoderma : Trichoderma Water control 0.284 0.0239 Tiller height (cm – on a log scale) Bacillus : Bacillus Water control 0.222 0.0002 Bacillus : Trichoderma Water control 0.193 0.0016 Bacillus : Water Water control 0.181 0.0038 Trichoderma : Bacillus Water control 0.269 0.0000 Trichoderma : Trichoderma Water control 0.183 0.0032 Trichoderma : Water Water control 0.179 0.0045 Days to 30% flowering (on a log scale) Bacillus : Water Trichoderma : Bacillus 0.144 0.0098 Trichoderma : Trichoderma Trichoderma : Bacillus 0.131 0.0256 Trichoderma : Water Trichoderma : Bacillus 0.155 0.0039 Fungicide Bacillus : Bacillus 0.179 0.0005 Fungicide Bacillus : Trichoderma 0.193 0.0001 Fungicide Bacillus : Water 0.130 0.0285 Fungicide Trichoderma : Bacillus 0.274 0.0000 Fungicide Trichoderma : Trichoderma 0.142 0.0110 Fungicide Trichoderma : Water 0.119 0.0614 Water control Bacillus : Bacillus 0.148 0.0010 Water control Bacillus : Trichoderma 0.163 0.0002 Water control Trichoderma : Bacillus 0.243 0.0000 Water control Trichoderma : Trichoderma 0.112 0.0296 Number of seeds per pedicle (on a log scale) Bacillus : Trichoderma Fungicide 0.200 0.0022 Trichoderma : Bacillus Fungicide 0.209 0.0011 Bacillus : Bacillus Water control 0.248 0.0000 Bacillus : Trichoderma Water control 0.324 0.0000 Bacillus : Water Water control 0.200 0.0002 Trichoderma : Bacillus Water control 0.333 0.0000 Trichoderma : Trichoderma Water control 0.203 0.0002 Trichoderma : Water Water control 0.206 0.0001 Shelled yield (kg per plot on a log scale) Bacillus : Trichoderma Bacillus : Water 0.460 0.0086 Trichoderma : Bacillus Bacillus : Water 0.583 0.0003 Bacillus : Trichoderma Trichoderma : Water 0.545 0.0009 Trichoderma : Bacillus Trichoderma : Water 0.669 0.0000 Bacillus : Bacillus Fungicide 0.514 0.0021 Bacillus : Trichoderma Fungicide 0.737 0.0000 Trichoderma : Bacillus Fungicide 0.861 0.0000 Trichoderma : Trichoderma Fungicide 0.518 0.0019 Bacillus : Bacillus Water control 0.882 0.0000 Bacillus : Trichoderma Water control 1.105 0.0000 Bacillus : Water Water control 0.646 0.0000 Trichoderma : Bacillus Water control 1.229 0.0000 Trichoderma : Trichoderma Water control 0.886 0.0000 Trichoderma : Water Water control 0.560 0.0000 Fungicide Water control 0.368 0.0202 Incidence of grains with blast symptoms (on the logit scale) Bacillus : Water Bacillus : Trichoderma 0.854 0.0030 Bacillus : Water Trichoderma : Bacillus 0.683 0.0350 Trichoderma : Water Bacillus : Trichoderma 0.703 0.0266 Water control Bacillus : Bacillus 1.250 0.0000 Water control Bacillus : Trichoderma 1.580 0.0000 Water control Bacillus : Water 0.726 0.0037 Water control Trichoderma : Bacillus 1.409 0.0000 Water control Trichoderma : Trichoderma 1.061 0.0000 Water control Trichoderma : Water 0.877 0.0002 Water control Fungicide 1.012 0.0000 The average final height was 34.1 cm (± 1.09). The final plant height differed (p < 0.001) among the eight treatments: all six microbial treatments increased plant height over the water control (Table 2). Foliar spraying with Bacillus led to higher plants than Trichoderma (p < 0.05); there were no synergies in alternate use of Bacillus and Trichoderma.
Days to 30% flowering and harvest
-
On average, it took 66.9 d (± 1.20) to reach 30% flowering. The time to 30% flowering differed (p < 0.001) among the eight treatments, due primarily to the fact that microbial treatments reduced the time to 30% flowering (Fig. 4; Table 2). For instance, the time to 30% flowering for the water control was 73.9 d (± 1.63), compared to 67.7 d (± 2.45) for dipping only treatments. Applying microbial products as foliar spray at the booting stage also reduced (p < 0.01) the time to 30% flowering, which was due primarily to the synergies in alternate use of Bacillus and Trichoderma (Fig. 5): time to 30% flowering was 60.6 d (± 1.95) for the two treatments of using the two products in alternative, compared to 65.5 d (± 2.51) for the two treatments using the single products as dipping and foliar spray. The average time to grain maturity was 95.5 d (± 1.42). The treatment effects on the time to maturity were similar to, but less pronounced than, those on the time to 30% flowering.
Figure 4.
Number of days to 30% flowering of rice plants that had received water/fungicide or one of the two biopesticides as dipping at transplanting only, or both as dipping and as foliar spray at three sites in 2022 in Tanzania. The bar represents one standard error, and P value is associated with the F-test in ANOVA, indicating the overall differences among the nine treatments.
Figure 5.
Net grain yield (kg) per plot (size 5 m × 5 m) of rice plants that had received water/fungicide or one of the two biopesticides as dipping at transplanting only, or both as dipping and as foliar spray at three sites in Tanzania in 2022. The bar represents one standard error, and P value is associated with the F-test in ANOVA, indicating the overall differences among the nine treatments.
Yield
-
Overall, there were 107 seeds (± 1.91) per panicle. The eight treatments differed (p < 0.001) in the number of seeds per panicle, due primarily to the fact that the water/fungicide control had fewer seeds than microbial treatments (Table 2). Foliar spraying led to further increases (p < 0.01) in the number of seeds per panicle, due primarily to the synergies in alternate use of Bacillus and Trichoderma: 88.8 seeds (± 1.95) (water control), 108 seeds (± 2.62) (dipping only), 111 seeds (± 2.76) (dipping + spray with the same product), and 122 seeds (± 1.56) (alternate use of the two products). This synergy was highly significant (p < 0.001).
On average, the net grain yield was 3.13 kg (± 0.167) per plot. There were significant (p < 0.001) differences among the eight treatments (Fig. 5), accounting for 72.9% of the total observed variability. The water control had a lower yield than all the other treatments, and the fungicide treatment had a lower yield than the four treatments with microbial products applied both at dipping and post-transplanting (Table 2). Foliar spraying led to further increases (p < 0.001) in the net grain yield, and there was a significant (p < 0.01) synergy from alternate use of the Bacillus and Trichoderma products. The average net grain yields achieved were 1.51 kg (± 0.123) (water control), 2.77 kg (± 0.210) (dipping only), 3.56 kg (± 0.195) (dipping + spray with the same product), and 4.70 kg (± 0.227) (alternate use of the two products). Overall, the two Bacillus and Trichoderma products did not differ in grain yield when applied as a dipping or foliar spray.
Blast development
-
On average, the incidence of grain with blast symptoms was 16.1% (± 1.20%). There were significant (p < 0.001) differences among the eight treatments (Fig. 6), accounting for 57.9% of the total observed variability. The water control had a higher blast incidence than the other treatments and the six microbial treatments did not differ in the blast incidence from the fungicide control (Fig. 6 & Table 2). Foliar spraying led to further reductions (p < 0.001) in the blast incidence, and there was a significant (p < 0.05) synergy in blast control from alternate use of the two Bacillus and Trichoderma products. The incidences of grains with blast were 28.9% (± 1.94%) (water control), 15.5% (± 1.62%) (dipping only), 11.4% (± 1.36%) (dipping + spray with the same product), and 8.49% (± 1.24%) (alternate use of the two products). Overall, the two Bacillus and Trichoderma products did not differ in the blast incidence when applied as dipping or foliar spray.
Figure 6.
Percent grain with blast symptom in each plot of rice plants that had received water/fungicide or one of the two biopesticides as dipping at transplanting only, or both as dipping and as foliar spray at three sites in Tanzania in 2022. The bar represents one standard error, and P value is associated with the F-test in ANOVA, indicating the overall differences among the nine treatments.
Yield increase achieved by the two microbial products was unlikely due entirely to the reduced blast development. All the six microbial treatments did not differ in the blast incidence from the fungicide control, but four microbial treatments where microbial products were applied both as dipping and foliar spray resulted in higher yields than the fungicide control (Table 2). Figure 7 shows grain blast incidence plotted as against grain yield for individual plots. Many microbial-treated plots had much higher yields than the fungicide-treated plots although the blast incidence was similar.
-
We extended our previous research to assess the effects of using commercial microbial products on rice blast and yield in Tanzania (low yield region - subsistence agriculture) and Kenya (relatively high yield and irrigated paddy rice production region). Low yield obtained in Tanzania, compared to that in Kenya, may have resulted from several factors, including variety genetic potential, blast development (higher in Tanzania), soil fertility, irrigation (used in the Kenya) and climatic conditions. Using microbial products led to significant reductions in blast development with the control efficacy similar to the fungicide treatment. In addition to agreeing with the previous study[18] on the increased yield due to microbial products in the subsistence farming region, the present results demonstrated that alternate use of B. subtilis and T. asperellum at transplanting (as dipping) or post-transplanting (as foliar spray) led to further yield increase (ca. 32.2%, 457 kg per ha) over the use of a single microbial product over time. Alternate use of B. subtilis and T. asperellum resulted in about 123.8% (1.04 ton per ha) and 211.0% (1.28 ton per ha) increase in grain yield over the fungicide and the water controls, respectively. Comparison with the fungicide control suggested that increased yield resulted mostly from improved plant development rather from reduced blast incidence.
All microbial treatments led to significant reductions in the blast incidence and achieved an efficacy similar to the fungicide control, although the blast incidence was lower than previous studies at the same site[18]. This agrees with previous studies demonstrating that other B. subtilis and Trichoderma strains can reduce rice blast development[14−16,21]. The blast fungus can colonise roots and lead to systemic invasion and classical disease symptoms on the above-ground plant parts[22]. In addition to direct competition with the blast fungus in the roots, root dipping and seed-coating may affect the pathogen via their effects on rhizosphere and endophyte microbiome. However, we recently demonstrated that rhizoplane and root endophytes of rice seedlings were not much affected by dipping in either B. subtilis Bs01 or T. asperellum T-900 (Xu, unpublished). Reduced blast development could also have resulted from the indirect effect through plant defence responses induced by the applied beneficial strains. Numerous studies have demonstrated that plant defence responses induced by Bacillus or Trichoderma spp. led to improved plant tolerance or resistance against specific pathogens and/or improved plant development[23−28].
Reduction in blast development is, however, not necessarily equivalent to yield gains, especially for low yielding sites in Tanzania: several microbial treatments led to much greater yield than the fungicide control although the blast incidence was similar. At low yielding sites, applying microbial products led to large increases in grain yield as demonstrated previously[18], due primarily to the increased number of tillers per plant and grains per panicle. In addition, microbial treatment, particularly dipping at the transplanting stage, shortened the time to flowering and maturity. Thus, we speculate that increased yield is due largely to improved plant development induced by applied microbes, rather than due directly to reduced blast development. Even at the high yielding site in Kenya, microbial treatments led to an overall significant increase (ca. 20%) in grain yield over the untreated control. However, as the microbial treatments had similar levels of blast development and grain yield as the fungicide control at the Kenya site, it is not possible to exclude the possibility that the yield increase associated with microbial treatments is due entirely to reduced blast development.
The three formulated products (B. subtilis, T. asperellum and S. nematodiphila strains) did not differ significantly in terms of rice blast development and grain yield at the Kenya site when applied as dipping at transplanting or post-transplanting foliar spray. Similarly, the B. subtilis and T. asperellum products did not differ significantly at three sites in Tanzania. Overall, these results are consistent with our previous study[18] although the previous study did show some-site specific differences between B. subtilis and T. asperellum products in Tanzania. Moreover, seed-coating with one specific S. nematodiphila strain led to a similar effect as transplanting dipping with or without foliar spray. In addition to induced host defence responses, seed-coating may also influence rhizosphere microbiome (as with dipping) that may affect nutrient uptake. Coating seeds with beneficial microbes is an efficient system to deliver beneficial microbes for improving seed germination and seedling establishment[6,7]. Efficacy of seed priming or dipping seedlings with beneficial microbes have been demonstrated in controlled conditions to suppress rice blast development, including B. subtilis and Trichoderma strains[14−16,21]. In the present study, we demonstrated the positive effect of seed-coating with beneficial microbes on rice productivity under commercial production conditions.
Additional foliar spray following a transplant dipping treatment with the same product did not lead to any additional benefit in Kenya. In contrast, additional spray with the same product as for dipping led to additional yield increases (average 313 kg per ha) in Tanzania, though not as much as increases (average 504 kg per ha) achieved by the dipping only treatments. As we argued above, increased yield is likely due primarily to induced plant responses. Thus, additional foliar applications of the same products as used in dipping (around 6-9 weeks after transplant dipping) may have strengthened plant development pathways previously induced by dipping. Such strengthened plant responses may be more important for plants grown under more stressful conditions. The Kenya experiment was conducted in the irrigation area. In contrast, the three Tanzanian experimental sites relied on rain-fed water, and thus plants may have experienced some drought stress at these sites. Indeed, second season crops were planted in the same sites in order to repeat the trials, as we did in Kenya. However, these repeat trials failed to produce grains because of drought in late 2022 in Tanzania. The differing degree of abiotic stress between Kenya and Tanzania experimental sites may thus explain the differences in the effects associated with additional foliar sprays.
Variable biocontrol efficacies and consistencies have led to the suggestion of using multiple biopesticides simultaneously to exploit possible synergies among biopesticides and hence improve performance as well as consistencies. However agonistic interactions between component microbes have often been observed, and thus synergistic interactions among biopesticides have rarely been achieved[19]. Our previous study[18] demonstrated antagonistic interactions between B. subtilis and T. asperellum when used in a mixture as dipping or foliar spray. Thus, in the present study, we investigated alternate use of B. subtilis and T. asperellum products over time for their effects on rice productivity. To our surprise, alternate use of the two products consistently outperformed the use of single products, leading to an average 32.2% increase in grain yield (458 kg per ha) over the single products. This additional benefit resulting from the alternate use of the two products may have resulted from the fact that the two (B. subtilis and T. asperellum) strains do not induce the same host responses either qualitatively (i.e., inducing different pathways) or quantitively (i.e. same pathways but induced to a different degree). Thus, applying the two products alternately over time may lead to a more complete plant response. Strains from B. subtilis and Trichoderma spp. can induce systemic defence response on many plant species[23, 26−31]. When applied in a mixture, direct competition between the two strains may have weakened the induced plant response. In addition, plant responses at a given time might be limited and hence may not be able to fully respond to the simultaneous use of the two strains. To quantify and statistically test the synergy in the alternative use of the two strains is difficult because the exact definition of synergy (hence statistical test) depends on the extent of overlap in induced plant responses by the two microbes and also dose-response relationships[19].
In summary, all microbial treatments led to significant reductions in rice blast and increased rice grain yield, particularly in the low yielding region in Tanzania. Alternate use of formulated B. subtilis and T. asperellum products at transplanting (as dipping) or post-transplanting (as foliar spray) led to additional yield increase in the low yielding region over the use of a single organism product at both times. Seed coating with one Serratia strain led to blast control and grain yield comparable to applying microbial products as transplant dipping and post-transplanting foliar spray as well as the fungicide control. Thus, present research results support the use of microbial products, particularly alternative use of different microbial products over time, to improve rice productivity in subsistence farming. Future research is needed to exploit potential benefits of combining seed coating with transplant dipping and/or post-transplanting foliar spray.
This research is funded by Innovate UK (project number 105665).
-
Xu X and Robinson-Boyer L declare no conflict of interest; Murunde R and Ringo G are employed by Real IPM who produced the three commercial biopesticides evaluated in the present study. The funders had no role in the design of the study; in the collection, analyses, or interpretation of data; in the writing of the manuscript; or in the decision to publish the results.
- Copyright: © 2023 by the author(s). Published by Maximum Academic Press, Fayetteville, GA. This article is an open access article distributed under Creative Commons Attribution License (CC BY 4.0), visit https://creativecommons.org/licenses/by/4.0/.
-
About this article
Cite this article
Murunde R, Ringo G, Robinson-Boyer L, Xu X. 2023. Applying beneficial microbes as transplanting dipping and post-transplanting foliar spray led to improved rice productivity. Technology in Agronomy 3:7 doi: 10.48130/TIA-2023-0007
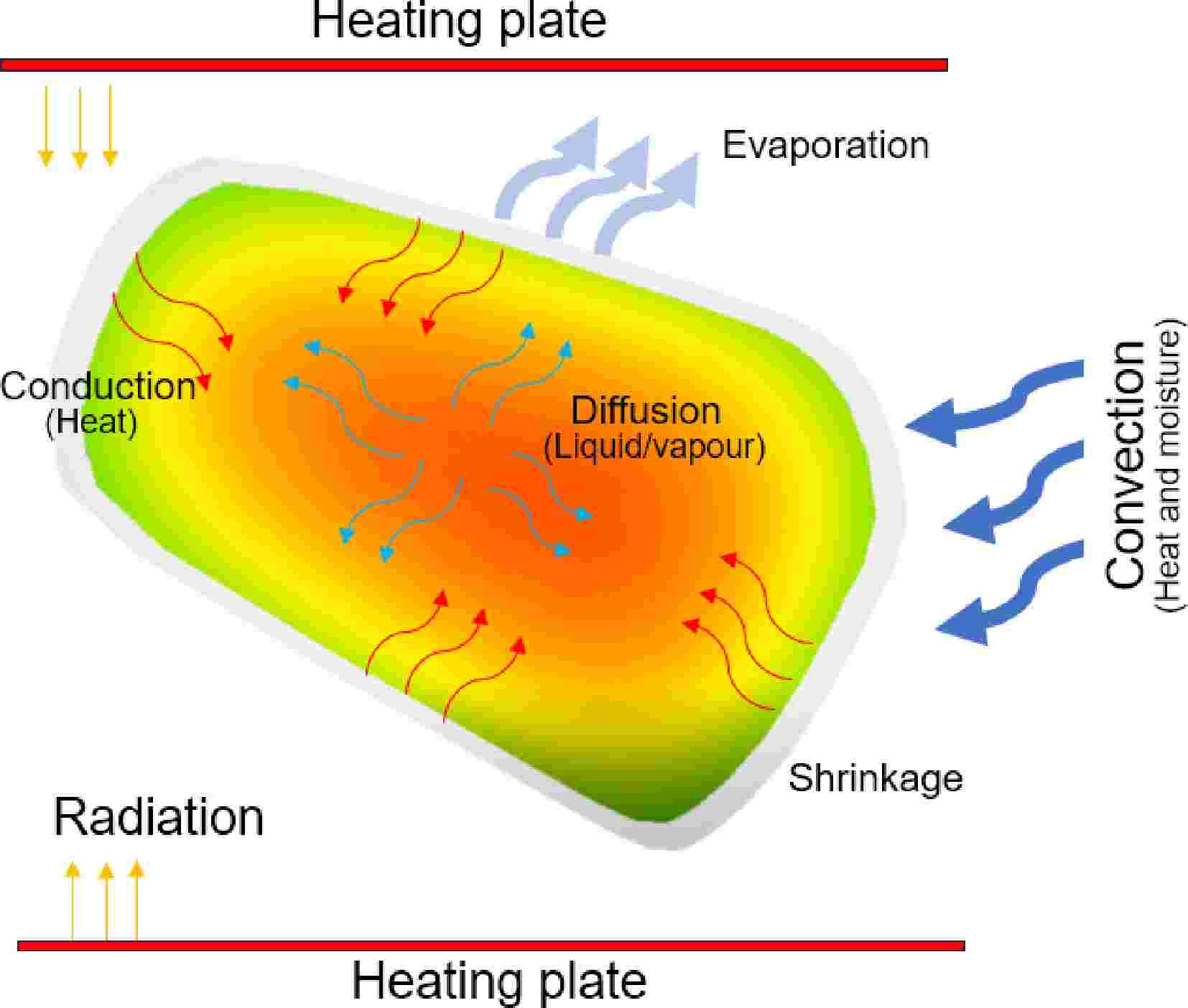