-
Carnation (Dianthus caryophyllus L.) is a perennial herb and has become a famous cut flower plant because of its vivid flower color, beautiful flower shape, strong fragrance, long vase life, and wide variety of horticultural species[1]. It is widely cultivated around the world by artificial cultivation, and in China it is mainly distributed in Fujian, Hubei, Yunnan and Ningxia. When the cut flowers of carnation are harvested, the water supply of the parent plant is cut off, producing water stress, increased microorganisms, blocked ducts, bacteria multiplying in water, and disrupted cuttings, which eventually affects the water absorption rate of the plant. This leads to impaired ornamental quality of cut flowers. Studies have shown that the lifespan of the cut flowers of carnation is twice as long when the water is close to saturation than when only 80% water content is available.
Ethylene has an important role in fruit ripening as well as flower fertilization, senescence and organ abscission[2−4], and in addition to its role in plant growth and development, ethylene is an important component of the response to biotic and abiotic environmental stresses[5−8]. Carnation is highly sensitive to ethylene, with 24 h exposure to 1−3 ppm ethylene causing severe damage. Recent studies indicate how ethylene regulates carnation petal senescence[9−18]. Flower senescence in most carnation varieties is characterized by autocatalytic ethylene production and petal wilting[19], but ethylene production and postharvest vase life vary among different carnation cultivars, and flowers of some longer-lived vase varieties do not correlate with reduced, delayed ethylene production[20],and their response to the degree of response to ethylene also depends on varietal differences[21]. Some varieties with longer vase life also exhibited higher ethylene sensitivity, while some varieties with shorter vase life exhibited lower ethylene sensitivity[22].
There are two rate-limiting steps in the ethylene biosynthesis pathway: S-adenosylmethionine (SAM) produces 1-aminocyclopropane-1-carboxylic acid (ACC) via ACC synthase (ACS), followed by the conversion of ACC to ethylene via ACC oxidase (ACO)[23], Thus ACS and ACO are considered to be rate-limiting enzymes in the ethylene biosynthetic pathway, encoded by the genes ACS and ACO, respectively. Three genes encoding ACS (DcACS1, DcACS2 and DcACS3) and one gene encoding ACO (DcACO1) have been identified in carnation. In fact, ethylene production was inhibited in petals of carnation strains transformed by the DcACS1 gene[24−29]. The expression levels of ethylene biosynthesis genes in different carnation varieties were negatively correlated with flower longevity, while the expression levels of DcACS and DcACO genes and ethylene production were lower in the pistil of varieties with delayed petal senescence and extended longevity[30]. The reason why the expression levels of DcACS and DcACO genes remain low during flower senescence in long-lived varieties has not been explained, but it may be that they have DcACS and DcACO alleles, the regulation of which is disturbed during natural senescence. Relatively high concentrations of ethylene (10 ppm) induced the expression of DcACS and DcACO genes in long-lived varieties, whereas expression was induced at lower ethylene concentrations in short-lived varieties[31]. The antisense cDNA of the ACO gene was introduced into carnation to obtain carnation strains with significantly delayed senescence. It has been found that overexpression of antisense sequences of the ACO gene in carnation delayed the onset of petal wilting while suppressing ethylene production in the transgenic carnation system to negligible levels by targeting the ACO gene, resulting in longer cut flower life[32].
There is still a lack of reports on the postharvest physiological changes of different species of carnation, therefore, this study illustrates the sensitivity of different species of cut flowers of carnation to ethylene as well as the changes in water content during senescence and the changes in the release pattern of endogenous ethylene, and further elaborates the molecular mechanism of ethylene regulation of senescence of cut flowers of carnation through molecular regulation.
-
The test selected 'Prince', 'Pink Diamond', 'Beit', 'Mallow Titanium Alloy', 'Hongfu', 'Seashell', 'Flame', 'Snow White', 'Little Pink', 'Master', 'Cloud Shium', 'Freedom', 'Deli' and 'Carnival', 14 varieties were tested, purchased from Colorful Flowers, all in the bud stage. Immediately after transport to the laboratory, they were rehydrated for 2 h. After rehydration, the bottom 3−5 cm were cut off diagonally in water, retaining the flowering stem length of about 50 cm and three pairs of leaves.
Record of vase life
-
For the ethylene treatment of the above carnation species, the cut flower stems of carnation were cut to a length of 15 cm and placed in a 100 mL conical flask containing deionized water, and the conical flask was placed in a 300 mm diameter glass desiccator with a 1 mL syringe to slowly inject 0.1 mL of ethylene gas at a concentration of 10 uL/L into the desiccator for 4 h. The deionized water treatment was used as the control group. Bottling was performed in conical flasks with a capacity of 100 mL and 50 mL of bottling solution (deionized water), which was changed every other day. Four flowers per treatment were observed at 20:00 each day, and the vase life was recorded by photograph.
Determination of ethylene release
-
Weighing and recording of potted carnation flowering branches, and then the flowers to be tested were placed in a sealed 50 mL vase containing 10 mL of distilled water and sealed for 6 h. The endogenous ethylene release was measured by GC-2010 gas chromatograph. One mL of gas was extracted from the sealed vial and injected into the gas chromatograph using a GDX-502 column with an inlet temperature of 130 °C, a pressure of 12 psi, a spacer purge flow rate of 3 mL/min, a column chamber temperature of 90 °C, an equilibration time of 1 min, a shunt ratio of 1:1, helium (99.999 %) as the carrier gas, a holding time of 3 min, and a detector temperature of 220 °C. Detector flow rate: tail blow gas flow rate (N2) 5 mL/min, reference flow rate 10 mL/min. Then the amount of ethylene released (ng/g·h) was calculated based on the volume of the sealed vial, sample weight and ethylene treatment time. Three flowers per treatment were measured and replicated three times.
Determination of water content
-
The sample petals were accurately weighed to 2 g, laid flat in a ceramic evaporating dish dried to a constant weight at 100−105 °C for 6 h, transferred to a desiccator, cooled for 30 min, weighed precisely, dried again at the above temperature for 1 h, cooled, and weighed until the difference between two consecutive weighing did not exceed 5 mg. The water content (%) in the sample was calculated according to the weight loss.
Quantitative real-time PCR (qRT-PCR)
-
Total RNA was extracted from petals and discs of different varieties under different ethylene treatment times using TransZol UP kit HiScript® II (Novozymes), and reverse transcribed using QRT SuperMix for qPCR reverse transcription kit (Novozymes) to determine the expression of DcACS1, DcACO1 and DcSAG12. The reaction is a 10 uL system: Hieff® qPCR SYBR® GREEN MasterMix is 5 uL, diluted cDNA is 3 uL, primer F and primer R is 1 uL each. Reaction program is: pre-denaturation, 95 °C, 5 min, 1 cycle; denaturation, 95 °C, 10 s, 40 cycles; annealing, 60 °C, 20 s, 40 cycles; extension, 72 °C, 20 s, 0 cycles; lysis curve stage, instrument default setting, 1 cycle. 72 °C, 20 s, 40 cycles; dissolution curve phase, instrument default setting, 1 cycle[33]. The Ct values were determined and the relative expression of genes in each sample was calculated using 2−ΔΔCᴛ[34]. Three biological replicates were set up and three technical replicates were designed for each biological replicate. The data were analyzed using GraphPad Prism8 software, and the significance of differences was analyzed by t-test, and the Bar value indicates the standard deviation. The primers required for the reactions are shown in Supplemental Table S1.
Transient gene silencing and overexpression
-
Transient silencing and transient overexpression of Agrobacterium shamrocki were performed by combining Agrobacterium TRV1, Agrobacterium TRV2, Agrobacterium TRV2-DcACS1, Agrobacterium TRV2-DcACO1, Agrobacterium 35S:GFP, Agrobacterium 35S:DcACS1-GFP, and Agrobacterium 35S:DcACO1-GFP in 50 mL of LB containing Kan and Rif at 28 °C. Constant temperature shaker 200 r/min shaking overnight.
The bacterial solution was then collected under aseptic conditions and resuspended with resuspension solution to OD600 ≈ 1.5. TRV1 resuspension, TRV2 resuspension and were mixed 1:1 with TRV2-DcACS1 resuspension and TRV2-DcACO1 resuspension, respectively, as well as 35S:GFP resuspension was mixed 1:1 with 35S:DcACS1-GFP resuspension and 35S:DcACO1-GFP resuspension, respectively, and left to stand for 3−4 h at 28 °C. The discs were punched on the outer petals with a puncher, placed into different bacterial solutions, put into the vacuum pump, and slowly deflated when the pressure reached 0.7 atm and kept for 5 min. After the infestation was completed, the flower discs were washed with distilled water two to three times and transferred to a dark environment at 8 °C for 3 d. Photographing and recording indicators. Sixteen discs per replicate and at least three biological replicates were performed. The required primers are shown in Supplemental Table S1.
Measurement of ion permeability
-
The samples of the floral discs collected at different time points were placed in 50 mL centrifuge tubes and 25 mL of 0.4 M mannitol was added. A constant temperature shaker at 28 °C at 200 r/min was shaken for 3 h. The initial value of conductivity X was measured by a conductivity meter. Then the centrifuge tubes were placed in an 85°C water bath for 20 min, and the total conductivity Y was measured after natural cooling. The relative conductivity was calculated as the initial value X/total conductivity Y. Sixteen pieces of each sample disc were taken and the measurement was repeated three times. The t-test was used to analyze the significance of differences.
Data processing and statistical analysis
-
Data were analyzed and organized using Excel 2019, and data were analyzed by ANOVA (p < 0.05) using SPSS23 statistical analysis software, and hypothesis ANOVA test using S-N-K method. GraphPad prism8 software was then used to create graphs.
-
Variations in vase life and sensitivity to ethylene have been reported for different carnation varieties, but no detailed analysis has been performed so far. Thus, 14 commercial carnation varieties with high economic and ornamental values were ordered and treated with ethylene gas at a concentration of 10 μL/L for 4 h for comparison with the vase life in the natural state. As shown in Fig. 1 and Table 1, the differences in the life span of these 14 carnation varieties in the natural vase stage and after ethylene treatment were observed. The shortest vase life in the natural state was 'Prince', and the longest vase life was 'Carnival'. The shortest vase life after ethylene treatment was 'Master', and the longest vase life was still 'Carnival'.
Figure 1.
Carnation variety difference chart of ethylene treatment and natural state of vase days. (a) 'Prince'; (b) 'Pink Diamond'; (c) 'Beit'; (d) 'Mallow Titanium Alloy'; (e) 'Hong Fu'; (f) 'Seashell'; (g) 'Flame'; (h) 'Snow White'; (i) 'Little Pink'; (i) 'Master'; (k) 'Cloud Shium'; (l) 'Freedom'; (m) 'Deli'; (n) 'Carnival'. For each variety, the first row of flowers are natural and the second row of flowers are ethylene treatment. Scale bar = 5 cm.
Table 1. Vase life of different varieties of cut carnations.
Varieties Vase life (d) Natural state Ethylene treatment 'Prince' 8 ± 0.8d 6 ± 0.7cd 'Pink Diamond' 8 ± 0.8d 7 ± 1.2cd 'Beit' 8 ± 1.5d 7 ± 1.5cd 'Mallow Titanium Alloy' 9 ± 1.1cd 7 ± 0.8cd 'Hong Fu' 9 ± 0.8cd 7 ± 1.8cd 'Seashell' 10 ± 1.4cd 6 ± 2.2cd 'Flame' 12 ± 0.8bc 9 ± 1.4c 'Snow White' 12 ± 0.8bc 12 ± 0.8b 'Little Pink' 14 ± 0.9ab 8 ± 0.8c 'Master' 15 ± 0.8a 4 ± 0.8d 'Cloud Shium' 16 ± 3.6a 8 ± 1.6c 'Freedom' 16 ± 0.8a 13 ± 2.2ab 'Deli' 16 ± 1.8a 14 ± 1.4ab 'Carnival' 17 ± 2.1a 15 ± 1.2a The data in the chart are the mean ± standard deviation, different lowercase letters represent significant differences at the p < 0.05 level. In order to analyze the differences in ethylene sensitivity of these varieties, the difference in the number of days of bottling between ethylene treatment and natural state was studied. From Table 1, it can be seen that 'Snow White' had the same number of days in vase in the natural state and after ethylene treatment (both were 12 d) and there was no difference. From Fig. 2, it can be seen that among the 13 varieties with differences in vase days, 'Master' had the largest difference in vase life between ethylene treated and untreated (11 d), followed by 'Cloud Shium' (8 d) and 'Little Pink' (6 d), respectively. While the difference between the two treatments for the green variety 'Seashell' was 4 d, 'Freedom' and 'Flame' naturally aged longer than 3 d longer vase life after ethylene treatment. After being treated with ethylene, the vase life of 'Prince', 'Mallow Titanium Alloy', 'Hongfu', 'Deli' and 'Carnival' has been shortened by 2 d, while 'Pink Diamond' and 'Beit' have only been shortened by 1 d. So, it can be seen that among the 14 varieties, 'Master' has the highest ethylene sensitivity and 'Snow White' has the least sensitivity to ethylene.
Figure 2.
The vase life difference in days between ethylene treatment and natural state. Different lowercase letters indicate significant difference among different varieties (p < 0.05).
Differences in water content after ethylene treatment of different varieties of carnation
-
In order to investigate the water content changes during senescence, we measured the changes of water content during full bloom stage (FBS), beginning of wilting stage (BWS), half wilting stage (HWS) and complete wilting stage (CWS) in control and ethylene treated 'Master', 'Cloud Shium', 'Little Pink', 'Seashell', 'Freedom' and 'Snow White' cut flowers in vase, respectively. The changes in water content at the complete wilting stage (CWS). The water content of both control (CK) and ethylene treated (ETH) showed a decreasing trend with the senescence process; however, the rate of decrease in water content after ethylene treatment was significantly faster than that of the control, and the water content at all stages was significantly lower than that of the control (Fig. 3).
Figure 3.
Changes of water content in different varieties of carnation during senescence. (a) 'Master'; (b) 'Cloud Shium'; (c) 'Little Pink'; (d) 'Seashell'; (e) 'Freedom'; (f) 'Snow White'.
Differences in ethylene release from different species of carnation
-
Since the variation of vase life is caused by the difference of ethylene production, in order to investigate the reason for the different vase life of these six varieties, ethylene release was measured after treating six varieties of carnation cut flowers with ethylene for 0, 4, 8, 12, 24 and 48 h. As can be seen from Fig. 4, all six varieties had obvious jump peaks, except for 'Master', where the ethylene release first increased and then decreased, reaching a maximum at 24 h. The release of ethylene from 'Master' reached the maximum at 12 h, then decreased at 24 h and then increased slightly (Fig. 4a). The maximum ethylene release of 'Snow White' was only 1.80 ng/g·h (Fig. 4f), which was probably due to the low sensitivity of 'Snow White' to ethylene.
Figure 4.
Endogenous ethylene release from carnation at different time of ethylene treatment. (a) 'Master'; (b) 'Cloud Shium'; (c) 'Little Pink'; (d) 'Seashell'; (e) 'Freedom'; (f) 'Snow White'.
Differential expression of DcACS1, DcACO1, DcEBF1/2 and DcERF-1 in different carnation varieties
-
Real-time quantitative PCR analysis was used to analyze the expression patterns of DcACS1, DcACO1, DcEBF1/2 and DcERF-1 in different cut carnation cultivars. DcACS1 and DcACO1 are essential ethylene biosynthesis genes. In the six carnation varieties, the expression of DcACS1 and DcACO1 increased significantly with the increase of ethylene treatment time, especially in 'Master', which were 3418-fold and 171350-fold respectively, and 'Snow White' had the lowest fold increase in DcACO1 expression, which was only 4157-fold higher than the control. At the same time, the expression levels of DcACS1 in 'Cloud Shium' and 'Sea Shell' decreased at the late stage of ethylene treatment (Fig. 5).
Figure 5.
The relative expression of DcACS1 (a) and DcACO1 (b) in carnation at different times of ethylene treatment.
Moreover, DcEBF1/2 and DcERF-1 play an important role in ethylene signaling, DcEBF1/2 rapidly increased in response to ethylene treatment for 4 or 8 h and subsequently decreased, most notably in 'Master' (Supplemental Figs S1 & S2). However, the expression level of ERF was irregular, and only 'Master' and 'Little Pink' increased in response to ethylene during the initial stage of ethylene treatment (Supplemental Fig. S3). In summary, 'Master' is the most ethylene-sensitive variety.
Transient silencing of DcACS1 delays senescence in cut carnation flowers
-
To verify the biological functions of DcACS1 in carnation senescence, we constructed TRV-DcACS1 vectors to silence its expression in carnation flower discs using virus-induced gene silencing (VIGS) technique. Compared with the TRV control discs, DcACS1 exhibited reduced expression levels in TRV-DcACS1 silenced discs (Fig. 6b), and the phenotypes of the flower discs showed that the rate of disc whitening was reduced and senescence was less in the experimental group (Fig. 6a). Meanwhile, the conductivity level of the control group was significantly increased (Fig. 6c) and the expression level of DcSAG12 in the experimental group was significantly reduced compared with the control (Fig. 6d). And transient silencing of DcACS1 expression can also effectively prolong the lifespan of cut flowers of carnation under ethylene treatment (Supplemental Fig. S4).
Figure 6.
Silencing and overexpression of DcACS1 in 'Master'. (a) and (e) Change in phenotype on different days. (b) and (f) Relative expression of DcACS1. (c) and (g) Change of conductivity. (d) and (h) Relative expression of DcSAG12. * α = 0.05; ** α = 0.01; ***α = 0.001.
Transient overexpression of DcACS1 promotes senescence in cut carnation flowers
-
When the ethylene synthase gene DcACS1 was overexpressed in 'Master', there was a significant acceleration of senescence in cut flowers of carnation (Fig. 6e). There was a highly significant up-regulation of the relative expression of DcACS1 in the experimental group compared with the control (Fig. 6f). The conductivity of 35S:DcACS1-GFP was highly significantly increased compared with 35S:GFP (Fig. 6g). The relative expression of DcSAG12 was significantly increased in the experimental group compared with the control (Fig. 6h). It indicates that DcACS1 has the effect of promoting senescence in cut flowers. The overexpression of DcACS1 significantly shortened the lifespan of cut flowers of carnation.
Transient silencing of DcACO1 gene delays senescence in cut carnation flowers
-
To verify the function of DcACO1 gene, TRV-DcACO1 was transfected into Agrobacterium tumefaciens and then infested with petals of carnation for transient silencing of DcACO1. As shown in Fig. 7a, the discs of TRV in the control group senesced more than the experimental group. Figure 7b showed that the relative expression of DcACO1 in the control group was significantly higher than that of TRV-DcACO1. The conductivity results showed that the conductivity level of TRV-DcACO1 was significantly lower than that of the control group (Fig. 7c). In addition, the relative expression of DcSAG12 in the experimental group was significantly lower than that of the control group (Fig. 7d). And while ethylene treated carnation discs, transient silencing of DcACO1 still can prolong disc life (Supplemental Fig. S5).
Figure 7.
Silencing and overexpression of DcACO1 in 'Master'. (a) and (e) Change in phenotype on different days. (b) and (f) Relative expression of DcACO1. (c) and (g) Change of conductivity. (d) and (h) Relative expression of DcSAG12. * α = 0.05; ** α = 0.01; ***α = 0.001.
Transient overexpression of DcACO1 gene promotes senescence in cut carnation flowers
-
It can be seen from Fig. 7e that 35S:DcACO1-GFP senescence was more severe after overexpression of DcACO1 than the control. The relative expression of DcACO1 was highly significantly increased in 35S:DcACO1-GFP compared with the control (Fig. 7f). The conductivity results also showed a highly significant increase after overexpression of DcACO1 compared with the control (Fig. 7g). The relative expression of DcSAG12 was upregulated in 35S:DcACO1-GFP compared with the control (Fig. 7h). It further indicates that DcACO1 has the function of accelerating senescence in cut flowers of carnation.
-
Water metabolism is an important physiological process in fresh cut flowers after harvest. Dysregulation of water balance occurs when water loss has an effect on the normal physiological functions of cut flowers. Water imbalance is the main cause of senescence in cut flowers, so cut flower senescence is usually accompanied by water loss and a decrease in water content. It has been shown that ethylene treatment enhances the phosphorylation of Ser280 and Ser283 sites of PIP2;1 protein thereby increasing its water channel activity, leading to faster water loss in isolated leaves. On the other hand, ethylene treatment can trigger S280/S283 phosphorylation of PIP2;1 and increased water loss in plastid exosomes in the absence of PIP2;1/2 protein[35]. Therefore, when ethylene treated cut flowers of carnation showed significant water loss from petals, and the rate of water loss was faster and more than that of the control (Fig. 3).
In this study, we found that after treating 14 varieties of carnation cut flowers with ethylene gas at a concentration of 10 uL/L for 4 h, we found that 13 varieties of cut flowers showed accelerated senescence, wilting, accelerated color fading, and significantly shorter vase life. Among them, 'Master' had a shorter number of days in vase from 15 d to 4 d, while 'Snow White' did not differ significantly from the control after ethylene treatment (Fig. 1), indicating that 'Snow White' was the least sensitive to ethylene. The degree of ethylene sensitivity of 'Snow White' was the lowest. When the ethylene produced by cut flowers themselves reached a certain value, it would trigger a large amount of ethylene production, which would accelerate the senescence of cut flowers. The changes of ethylene production in cut flowers were classified into three types: ethylene leap-like, non-ethylene leap-like and terminal rise like. In the study of ethylene release of six varieties, it was found that 'Cloud Shium', 'Little Pink', 'Sea Shell', 'Freedom' and 'Snow White' belonged to similar ethylene leap type with obvious ethylene leap peaks, but relatively speaking, the ethylene leap peaks of 'Snow White' was gentle and the ethylene release was the smallest, which might be the reason why 'Snow White' was less affected by ethylene. The ethylene release of 'Master' belongs to a similar terminal ascending type, but the ethylene-sensitive type of 'Master' is the highest, which can also explain the complexity between ethylene and senescence in different varieties of carnation cut flowers (Fig. 4). This provides a good material to study the mechanism of senescence and ethylene in cut flowers of carnation, thus providing theoretical support to screen for long-lived carnation varieties in vase, but a lot of research is needed to really understand the association between senescence and ethylene in different varieties.
When ethylene is sensed by specific receptors, ethylene signals are sent through a series of biochemical events that regulate the expression of ethylene-responsive genes, leading to ethylene synthesis and ultimately to flower senescence[36]. In the present study, DcACO1 was detected to show the most pronounced expression pattern in ethylene treatment. In addition, the transcript levels of DcACO1 after ethylene treatment were strongly correlated with the degree of reduction in vase life in ethylene-sensitive and non-sensitive varieties. Changes in ethylene sensitivity may be related to the ability to sense or respond to ethylene, which is mediated by changes in ethylene signaling during flower development. It has been shown that the CTR immediately downstream of the ethylene receptor acts as a negative regulator in ethylene signaling and negatively correlates with ACS1 and ACO1 transcripts by mediating the mitogen-activated protein kinase module (MAPK)[37]. The results of the present study combined with those of previous studies indicated that among the ethylene biosynthesis genes, DcACO1 contributed the most to the changes in ethylene sensitivity in vanilla. In a study on the regulation of cut flower senescence by two key enzyme genes in the ethylene synthesis pathway, DcACS1 and DcACO1, it was found that DcACS1 and DcACO1 could promote cut flower senescence in carnation, and transient silencing of these two genes in 'Master' could significantly prolong vase life and accelerate cut flower senescence after overexpression of these two genes, which is consistent with the results of previous studies.
-
In this study, we investigated the ethylene sensitivity and postharvest changes of different carnation species, which laid the foundation for further research on the molecular mechanism of ethylene regulation of postharvest senescence of cut flowers of carnation, and also indicated the direction for further breeding and artificial screening of new storage tolerant carnation species by gene editing technology.
-
The authors confirm contribution to the paper as follows: study conception and supervision: Zhang F; study design: Min Wang, Ni C, Zhang F; experiment performance and data analysis: Min Wang, Man Wang, Ni C, Feng S, Wang Y, Zhong L; manuscript suggestions: Cheng Y, Bao M; draft manuscript preparation: Min Wang, Man Wang, Zhang F. All authors reviewed the results and approved the final version of the manuscript.
-
All data generated or analyzed during this study are included in this published article and its supplementary information files.
This work was supported by the Knowledge Innovation Program of Wuhan-Basic Research (Program No. 2023020201010106), the Fundamental Research Funds for the Central Universities (Program No. 2662023PY023 and 2662019PY049), the Thousand Youth Talents Plan Project and the Start-up Funding from Huazhong Agricultural University to FZ, and by the Fundamental Research Funds for the Central Universities (Program No. 2662023PY011 and Horti-PY-2023-001).
-
The authors declare that they have no conflict of interest. Fan Zhang is the Editorial Board member of Ornamental Plant Research who was blinded from reviewing or making decisions on the manuscript. The article was subject to the journal's standard procedures, with peer-review handled independently of this Editorial Board member and the research groups.
-
# Authors contributed equally: Min Wang, Man Wang, Chenyu Ni
- Supplemental Table S1 Primer sequences in this study.
- Supplemental Fig. S1 The relative expression of DcEBF1 in carnation at different time of ethylene treatment.
- Supplemental Fig. S2 The relative expression of DcEBF2 in carnation at different time of ethylene treatment.
- Supplemental Fig. S3 The relative expression of DcERF-1 in carnation at different time of ethylene treatment.
- Supplemental Fig. S4 Silencing of DcACS1 under ethylene treatment.
- Supplemental Fig. S5 Silencing of DcACO1 under ethylene treatment.
- Copyright: © 2024 by the author(s). Published by Maximum Academic Press, Fayetteville, GA. This article is an open access article distributed under Creative Commons Attribution License (CC BY 4.0), visit https://creativecommons.org/licenses/by/4.0/.
-
About this article
Cite this article
Wang M, Wang M, Ni C, Feng S, Wang Y, et al. 2024. Differences in ethylene sensitivity, expression of ethylene biosynthetic genes and vase life among carnation varieties. Ornamental Plant Research 4: e004 doi: 10.48130/opr-0024-0002
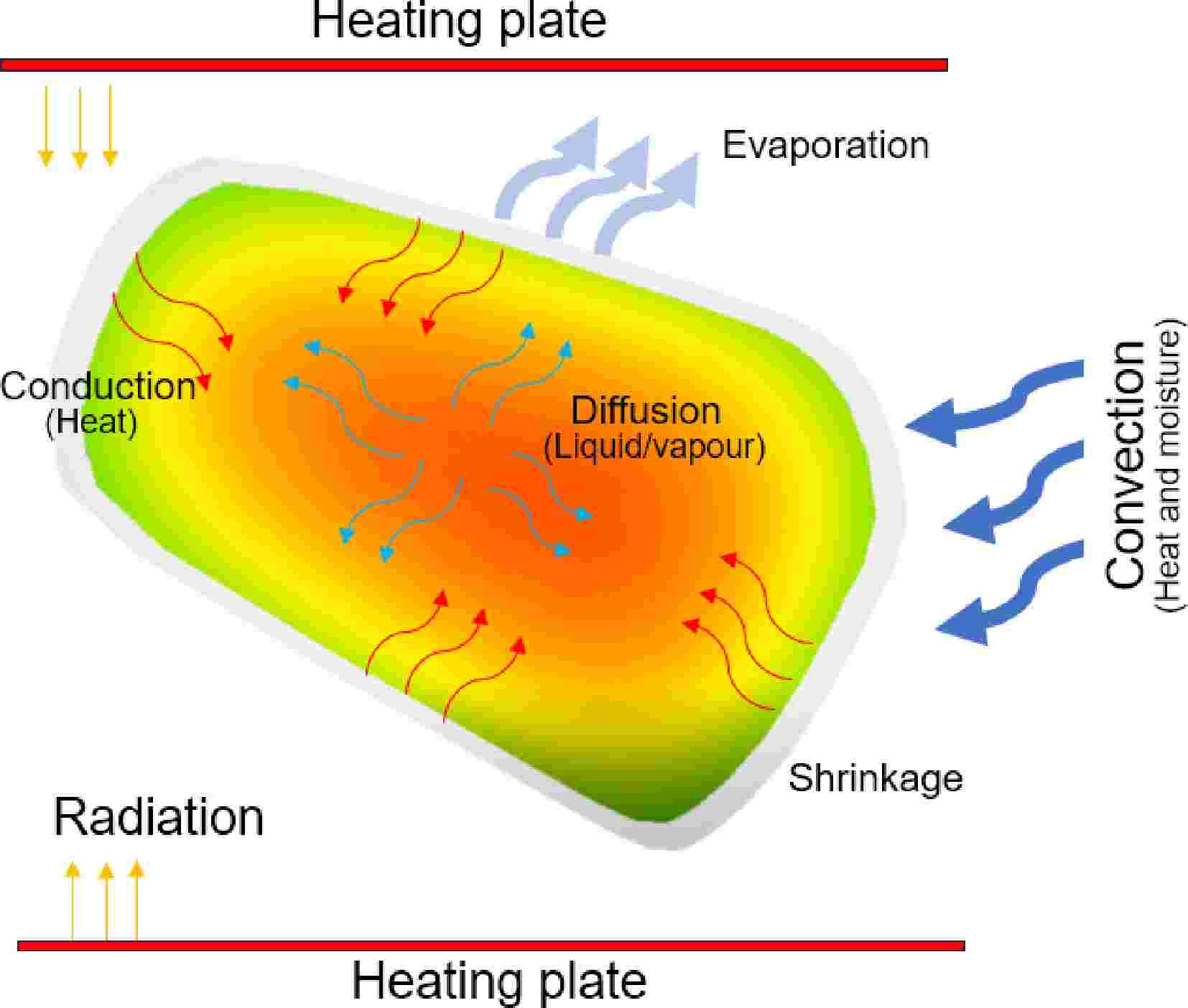