-
Nitrogen in the forms of urea, nitrates, and ammonium are essential compounds to living things providing a building block of many important basic biological compounds (such as amino acids, proteins, and deoxyribonucleic acid/ribonucleic acid nucleotides) required to grow tissue in animals and plants[1]. Nitrogen in its nonreactive dinitrogen (N2) form is the most abundant element in nature, constituting about 79% of total atmospheric air and 79.2% of soil air[2]. It was estimated that in fertile soil, the surface of 6 inches of an acre may contain about 35,000 tons of inert dinitrogen gas[3]. However, despite the large amount of nitrogen gas in the soil, it is unavailable to plants until it is converted to ammonium (NH4+) through reduction processes or nitrates (NO3−) through oxidation processes. There are three main processes involved in converting dinitrogen to plant-available forms; these include biological nitrogen fixation, lightning, and industrial nitrogen fertilizer production. Biological nitrogen fixation, a process by which microorganisms convert N2 into NH3 is restricted to only selected groups of plants (legumes) and some few free-living microorganisms[4], and contributes only about 21.45 Tg of fixed nitrogen per year, representing 42.9% of N fixed biologically in global agricultural systems[5]. Lightning, a process of an electric discharge that occurs during a thunderstorm also leads to the formation of nitrate when a nitrogen atom in the atmosphere is bonded to oxygen. However, this process is uncertain, and only produces 5 ± 3 Tg per annum LNOx globally[6].
The development of ammonia and nitrate synthesis has evolved significantly over the past century. The Haber-Bosch process, developed in the early 20th century, revolutionized ammonia production by enabling the fixation of atmospheric nitrogen under high temperature and pressure conditions using iron-based catalysts[7]. This involves reacting H2 and N2 at an elevated temperature (> 673 K) and pressure (> 200 bar) in the presence of a Fe-based catalyst to produce ammonia[8,9]. The discovery of this method in 1909 resulted in a massive increase in crop yield[10], promoting global crop production to nearly 400%, and enabling the world population to multiply four times[7]. However, the process is energy-intensive[11], uses about 1%–2% of the world's annual energy generation[12], and contributes around 3% of global greenhouse gas generation through the emission of a substantial amount of CO2 into the atmosphere[13,14]. Hence, the need for the development of an eco-friendly, sustainable, and environmentally benign method for mass ammonia synthesis.
An alternative and promising method for nitrogen fixation that is recently attracting attention is the photocatalytic N2 reduction to produce ammonia[12,15] and/or photocatalytic N2 oxidation to produce nitrate[16,17]. The process utilizes abundant solar energy, N2, and H2O under atmospheric pressure and ambient temperature in the presence of a photocatalyst. This process of nitrogen fixation is the most eco-friendly and sustainable method of nitrogen fixation.
-
Despite the abundance of nitrogen in the atmospheric and soil air, only a limited amount of reserved soil inorganic nitrogen in the forms of nitrate and ammonium is accessible to plants[18]. The limitation of nitrogen shortages in the soil was augmented for centuries through crop rotations with leguminous crops and the use of organic fertilizers like seaweeds, wood ash, and animal manures. Crop rotation mainly relies on biological nitrogen fixation where leguminous crops (with the aid of microorganisms) in rotation and free-living cyanobacteria help in converting atmospheric nitrogen to plant-available forms[4]. Apart from biological nitrogen fixation, another natural process of nitrogen fixation which is uncertain is lightning. It produces nitrate through the reaction of a nitrogen atom and an oxygen atom facilitated by an electric discharge in the atmosphere[6]. The highest and most important process of nitrogen fixation is the conversion of dinitrogen into ammonium on a large and industrial scale (Haber-Bosch process)[19]. These bring about the synthesis of mineral fertilizers which significantly increases crop production by 400%[20]. Artificially, a promising and alternative method of nitrogen fixation to the Haber-Bosch process is recently attracting much attention. This is photocatalytic nitrogen fixation using nanostructured materials[12,16]. Each of these processes has its advantages and disadvantages which will be reviewed in detail.
Natural processes of atmospheric nitrogen conversion
Biological processes
-
Biological nitrogen fixation (BNF) is a process whereby free-living and symbiotic bacteria convert atmospheric dinitrogen to ammonia in the global nitrogen cycle. The process accounts for almost two-thirds of the total natural fixed dinitrogen on the earth's surface and is facilitated by the action of the nitrogenase catalytic/enzymatic complex[21]. The microorganisms and the enzymatic routes that convert dinitrogen to different oxidative states are still not fully understood. Thus, to understand the various microorganisms and the enzymatic reactions, the major microbial processes and the major reactions responsible for the BNF were highlighted based on the present perspective.
Biological nitrogen fixation is a kind of ammonification reaction that is achieved with the help of archaea and bacteria[22]. The reaction requires a large amount of energy (16 ATP molecules) as shown in Eqns (1) and (2) below:
N2(g)+8H++8e−+16ATP→2NH+4+16ADP+16Pi (1) 2NH+4+2e−→2NH3+H2 (2) Among the microorganisms in the soil, only a few can use dinitrogen molecules, while others require a combined form of nitrogen[23]. The ammonium produced through the ammonification process is assimilated into biomass or utilized aerobically/anaerobically by ammonia-oxidizing microbes[24]. The ammonification process first produces ammonia in the soil. The ammonia then reacts with soil water to produce NH4+ ready for plant uptake[25,26]. Further to the BNF, the ammonia produced is also transformed into nitrite and nitrate[27]. The chemical reaction occurs via three steps (Eqns 3–5). The first step involves the action of monooxygenase (enzyme ammonia) on ammonia, catalyzing the oxidation of ammonia to hydroxylamine[28]. The second step is the conversion of hydroxylamine to nitrite with the aid of hydroxylamine oxidoreductase. The third step is the transformation of nitrite to nitrate in the presence of nitrite-oxidizing bacteria[29]. These processes are very important to plants because they produce more available nitrogen for plant uptake.
NH3+O2+2H++2e−→NH2OH+H2O (3) NH2OH+H2O→NO−2+5H++4e− (4) NO−2+1/2O2→NO−3 (5) The major drawback of BNF is that it only occurs in the nodules of the root of leguminous crops and/or by a few free-living archaea/bacteria in waterlogged/paddy conditions[30]. Even in leguminous crops, in the absence of root nodules or inefficiency of the nodulation, no nitrogen will be fixed. Also, the free-living bacteria that fix atmospheric nitrogen mostly do so in waterlogged/paddy conditions. Only a few crops of high value such as rice and cocoyam can grow in waterlogged conditions. Therefore, BNF cannot be depended upon for nitrogen fixation to meet the demands of the crops and to also feed the ever-increasing world population.
Atmospheric lightning
-
Lightning is a process of electric discharge with aural and visual effects accompanied by a lightning flash that occurs mostly during a thunderstorm. Although the process occurs in a relatively short period, it has intense chemical reactions which radically changed the chemical composition of the air in and around the discharge zone[31]. The electric discharge breaks the dinitrogen gas (N2) triple bond and combines them with oxygen to produce nitrogen dioxide (NO2) and nitrogen oxide (NO), or reacts with hydrogen to form ammonia as shown in Eqns (6)–(8)[32]. The oxides and ammonia further dissolve in precipitation (snow and rain) to yield nitrates (NO3−) and ammonium (NH4+) respectively which are deposited into the soil for plant assimilation.
N2(g)+O2(g)→2NO(g) (6) N2(g)+2O2(g)→2NO2(g) (7) N2(g)+3H2(g)→2NH3(g) (8) However, despite the many occurrences of lightning flashes around the globe, the process is not a reliable means of nitrogen compound production for plants, as it is highly uncertain and only produces 5 ± 3 Tg per annum NOx globally (5%–8% of fixed nitrogen)[6,33]. Therefore, lightning cannot be considered an alternative method of nitrogen fixation for sustainable crop production.
Artificial processes of atmospheric nitrogen conversion
Chemical processes
-
The Haber-Bosch process is a chemical fixation of nitrogen which involves reacting dinitrogen gas (N2) with hydrogen gas (H2) at very high temperatures (650–750 K) and high pressures (50–200 bar) in the presence of a Fe-based catalyst to produce ammonia (Eqns 9–14)[34,35]. The NH3 produced through this process is the key chemical component in the fertilizer industries with yearly production exceeding 150 million tons worldwide[36]. In addition, due to the high gravimetric density of hydrogen (17.75 wt%), ammonia is also used as a clean energy carrier for the chemical storage of energy[23].
N2(g)→N2(adsorbed) (9) N2(adsorbed)→2N(adsorbed) (10) H2(g)→H2(adsorbed) (11) H2(adsorbed)→2H(adsorbed) (12) N(adsorbed)+3H(adsorbed)→NH3(adsorbed) (13) NH3(adsorbed)→NH3(g) (14) In the Haber-Bosch method, the most significant and rate-determining step is the formation of atomically adsorbed nitrogen species through the dissociation of dinitrogen (Eqn 9), followed by hydrogen production which consumed the highest energy in the whole process. It involves steam methane reforming (SMR) at a high temperature of 800–1,000 °C (Eqn 15), and the purification of the effluents to remove CO and maximize H2 yield through water gas shift (WGS) exothermic reaction at 350–550 °C (Eqn 16)[37].
CH4+H2O→CO+3H2 (15) CO+H2O→CO2+H2 (16) There is no doubt that the discovery and industrial conversion of nitrogen through the Haber-Bosch process has transformed global crop production, however, the consequences attached to the development are of global concern. The purification of hydrogen in hydrogen production resulted in a massive release of carbon dioxide which contributes to around 3% of global greenhouse gas emissions[7]. Also, the process consumes a substantial amount of energy which is approximately 1%–2% of the annual global energy generation[12,38], and it only takes place at very high temperatures and pressure. Therefore, there is a need for the development of a sustainable and environmentally friendly method for nitrogen fixation.
Photocatalytic processes
-
Apart from the Haber-Bosch process, another artificial method of nitrogen fixation that is attracting much attention is photocatalytic nitrogen fixation to produce ammonia[12] and/or nitrate[16]. The process utilizes abundant solar energy, N2, and H2O under atmospheric pressure and ambient temperature in the presence of a photocatalyst. It is considered a substitute for the Haber-Bosch process[39,40], as it is the most eco-friendly approach for ammonia and nitrate synthesis[16,41]. The process involves light absorption by a photocatalyst (mostly nanostructured materials), usually a semiconductor with relatively low band gap energy, leading to electron excitation from the valence band moving to the conduction band, leaving behind positively charged holes in the lower valence band. In the presence of a trap, interfacial charge carrier transfer takes place which subsequently leads to the redox reaction where N2 is reduced to NH3 (Eqns 17–20).
2N2+6H2OPhotocatalyst→4NH3+3O2 (17) PhotocatalystLightabsorption→H++e− (18) 2H2O→4H+4e−1+O2 (19) N2+6H++6e−→2NH3 (20) The electrons in the conduction band have the potential to reduce N2 to NH3 utilizing the protons generated at the valence band where oxidation of H2O is taking place. Thermodynamically, the reaction possesses a Gibbs free energy of +339 kJ·mol−1 (endothermic reaction)[12].
-
Two reaction pathways result in photocatalytic nitrogen fixation. The first pathway of the reaction is the nitrogen reduction reaction (NRR) which results in the reduction of dinitrogen to produce ammonia[12], and the second is the nitrogen oxidation reaction (NOR) which results in the oxidation of dinitrogen to produce nitrate[16]. Both pathways involves the splitting of water molecules, breakage of dinitrogen triple bonds and the reduction/oxidation of dinitrogen to ammonia/nitrate respectively.
Splitting of water molecules
-
Water oxidation is the primary step of photocatalytic nitrogen fixation. The process is challenging and directly determines the fate of the reaction. Therefore, a photocatalyst with good water oxidation ability is most desirable.
The reaction of water splitting involves three major steps. The first step is band-gap excitation (photoexcitation) which results in the excitation of electrons generating electrons and holes inside the semiconductor. The second step is the movement of electrons into the conduction bands leaving behind holes within the valence band. Finally, the photogenerated electrons and the holes serve as the active sites for the redox reaction in which they are trapped and consumed by the catalytic water oxidation and reduction reaction[42]. In the reaction processes, the recombination of the photoexcited electrons with the holes occurs very fast, which is the main challenge of the processes. Therefore, for a successful redox reaction, the process must proceed within the lifetimes of the photoexcitation of the electrons and the hole generation[43]. Currently, strategies are being investigated for improving the lifetimes and trapping of photogenerated electrons for efficient redox reactions. These include the construction of heterojunction, doping technique, morphology engineering, spatial charge separation, and surface modification[44−46].
Breakage of N2 triple bond
-
Breaking the robust nitrogen-nitrogen triple bond is a pivotal step in both natural and artificial ammonia/nitrate synthesis[47]. The rate-limiting step of the reaction involves the cleavage of the nitrogen-nitrogen bond, forming surface-bound nitrides (N3−) (as illustrated in Eqn 9). This reaction demands a substantial amount of energy, approximately 945 kJ/mol, primarily due to the high ionization energy of the N2 triple bonds, measuring at 15.58 eV[48,49]. The robust interaction between the photocatalyst and the N 2p states leads to the chemical adsorption of N2 molecules on the outermost layer of the photocatalyst. This interaction establishes a conduit for electron transfer from the photocatalyst to N2, ultimately resulting in the cleavage and activation of nitrogen molecules into surface-bound nitrides (N3−). These surface-bound nitrides then undergo catalytic reactions, either with protons to generate ammonia or through oxidation to produce nitrate. Recent experimental data by Natarajan et al.[50] demonstrated that utilizing a titanium dioxide (TiO2) photocatalyst doped with transition metals significantly improved the nitrogen reduction reaction efficiency, achieving an ammonia production rate of 1.2 mmol·g−1·h−1 under solar irradiation. This finding underscores the potential of advanced photocatalyst modifications in overcoming the high energy barriers associated with nitrogen activation.
Reduction/oxidation of N2 to NH3/NO3−
-
In the NRR pathway, after the splitting of the water molecules generating electrons and the holes, and the breakage/activation of the N2, the hot electrons lead to the reduction of the N2 thereby producing NH3. For the NOR pathway, the photogenerated H+ oxidize N2 to NO with water while O2 is reduced to H2O by photoexcited electrons. Further, NO is finally oxidized to nitrate evolving O2 and H2O. In general, the whole processes rely on water and dinitrogen in the presence of a photocatalyst under ambient conditions with the help of sunlight as an energy source (Fig. 1). A recent study by Nabeel et al.[51] provided experimental evidence supporting this mechanism, where a modified g-C3N4 photocatalyst achieved a nitrogen reduction rate of 35.6 μmol·g−1·h−1 for NH3 production and 48.9 μmol·g−1·h−1 for nitrate under visible light irradiation. This study emphasizes the effectiveness of utilizing solar energy and advanced photocatalyst modifications to drive these reactions efficiently under ambient conditions.
-
Solar energy is primarily concentrated in the visible and infrared regions of the electromagnetic spectrum. This illuminates the earth and facilitates many important crop developmental processes such as photocatalytic nitrogen fixation, photosynthesis, seed germination, leaf expansion, general plant growth etc.
Nanomaterials are defined as a material having constituents/particles at nanoscale dimensions (1–100 nm) and generally possess electrical and optical properties emanating due to their size[52]. The first study on nanomaterial photocatalytic nitrogen fixation can be dated back to 1977 when Schrauzer & Guth[53] employed the Rutile phase of TiO2 nanomaterial as a catalyst to convert dinitrogen into ammonia under UV light. Later, many nanomaterials were reported to be active photocatalysts in photocatalytic N2 fixation, some of which include cadmium sulfides[54], diamond[55], cadmium zinc sulfide[56], carbon nitrides[57−59], titanium oxides[60], bismuth oxides[61], and many more. Although lots of successes were recorded in photocatalytic nitrogen fixation, many challenges affect the performance of the process. One of the most challenging parts is the splitting of the water molecules to provide the proton needed for the reduction of the dinitrogen. The thermodynamics of photocatalytic reactions is dictated by the conduction band minima and valence band maxima. The valence band maxima must have a potential higher than the redox potential of water oxidation. This remains one of the challenges associated with environmentally friendly photocatalysts such as graphitic carbon nitride (g-C3N4). Another challenging issue is the absorption of N2 molecule onto the surface of a photocatalyst and its activation by the photocatalyst as N2 molecule is chemically stable and requires high input energy (~941 kJ/mol) to activate them[12]. Also, the recombination of photogenerated carriers affects the quantum yield of N2-fixed due to the difficult diffusion of the photocatalyst and poor interfacial charge transfer[62]. Hence, a prerequisite for a material to simultaneously serve as a photocatalyst with high potential is its ability to oxidize water molecules and absorb and reduce/oxidize the N2.
Importance of nanomaterials in nitrogen fixation
-
Since the shape, size, and surface area are of great importance to the activities of nanomaterials, adequate attention should be given to their design and synthesis for efficient N2 fixation activity. Below are some of the properties of nanomaterials that make them unique in nitrogen fixation:
• Nanomaterials' reduced size leads to discrete conduction and valence bands, resulting in an expanded band gap (the energy difference between a nanomaterial's conduction and valence bands, which represents the energy range inaccessible to electrons in the material). Consequently, the conduction band becomes more negatively charged, while the valence band becomes more positively charged[63], enhancing the redox potential of nanomaterial photocatalysts.
• During the N2 redox reaction, the space charge layer's thickness typically surpasses the nanomaterials' particle size[64]. These distinctions result in significant variations between the electrical, magnetic, thermal, optical, and acoustic properties of nanomaterials compared to bulk materials[62]. This discrepancy enables photogenerated carriers to efficiently diffuse from the bulk phase to the surface, reducing migration distance and minimizing the likelihood of recombination. Consequently, higher redox advantages are achieved.
• Nanomaterials possess many active sites, defect densities and large specific surface areas. As the nanomaterials are on a nanoscale, this makes many atoms be exposed and a decrease in the size of nanomaterials increases the surface area greatly, thereby dramatically increasing the ratio of surface atoms to total atoms in the nanomaterials, translating to the surface atoms having high activity. These properties are conducive to aiding the separation of photogenerated carriers, N2 activation, and N2 adsorption, thereby improving the yield of the N2 fixation process.
Types of nanomaterials used in nitrogen fixation
-
The use of nanomaterials for photocatalytic N2 fixation is attracting much attention in recent times. Different types of nanomaterials have been produced to maximize their efficiency in N2 photofixation. The below sections discuss in detail the most notable advancements in nanotechnology for N2 photofixation.
Zero-dimensional (OD) nanomaterials
-
These are nanomaterials with negligibly small dimensions in which the dimensions are hardly visible using any known technique. Due to their possession of discrete energy levels, they are also called artificial atoms/quantum dots[65]. Examples include CdS, CdSe, CdTe, gold nanoparticles, silver nanoparticles, sulphur nanoparticles, etc. Among the different OD nanomaterials, sulphur nanomaterials are the most studied due to their versatile functionalities, simplistic synthesis, and excellent applications in the field of agriculture[66]. The main precursors for the synthesis of sulphur nanomaterials are Na2S2O3 and HCl and most synthesized nanomaterials possess a diameter of 0–50 nm. To effectively utilize OD sulphur nanomaterials for photocatalysis, different modification strategies were used. These include surface modifications, improvement of core and porous structures, production of thin films, nanohybrids, and assembly systems. This is to provide them with high colloidal stability and enhanced ability for a high-performance application.
One-dimensional (1D) nanomaterials (rods, tubes, wires, belts)
-
These are nanomaterials with two dimensions of particulates (thickness and width) on the nanoscale. They are in such a way that the third dimension (length) is significant compared to the other two dimensions which run for > 100 nm. 1D nanomaterials possess a good quality high aspect ratio which provides them with the property of accelerated separation and transport of photogenerated carriers, thus promoting photocatalytic nitrogen fixation activity. Many 1D nanomaterials were synthesized and their efficiency for nitrogen photofixation was determined. Among the notable ones are Bi5O7Br nanotubes[67]. It is self-assembled, 5 nm in diameter, does not have a sacrificial agent, possesses oxygen vacancies, and has a high specific surface area. Oxygen vacancies have been shown to capture and activate N2, and the high specific surface area provided them with many exposed active sites. Another important 1D photocatalyst is W18O49 ultrathin nanowires[68]. It is used as a model photocatalyst to promote oxygen vacancies using Mo doping. This is to provide many sites for electron transfer and N2 chemisorption and to also utilize the strong coordination between Mo and N atoms which enhances the adsorption and activation of N2[69]. In addition to the two photocatalysts discussed above, NiS/CdS nanorods[70] and MoO3-x nanotubes[71] have also been used with success and yielded relatively high nitrogen fixation.
Two-dimensional (2D) nanomaterials (sheets)
-
These are nanomaterials with only one dimension (thickness) in the nanoscale, and the other two dimensions (length and width) are significantly larger compared to the thickness. The 2D nanomaterials have attracted a greater interest for photocatalytic nitrogen fixation, due to their nano thickness, which gives out more exposed and defective surface-active sites, resulting in improved adsorption and activation of N2 molecules. Comparing the Bi3O4Br bulk and the Bi3O4Br nanosheet revealed that the nanosheet has 30.9 times higher nitrogen photofixation activity compared to the bulk[72]. A CuCr nanosheet with a thickness of 2.5 nm has expressed the highest NH3 evolution rate (142.9 μmol·L−1·h−1) in comparison to its bulk counterpart[73]. Furthermore, the nanosheets also proved to possess higher photoactivity compared to the bulk, as seen by ultrathin Cu-doped TiO2 which has 700 nm photoactivity that is by far compared to the bulk[74]. This result is attributed to the doping of Cu which increased the charge transfer between TiO2 and N2 and weakened the N2 triple bond. The higher activity of the 2D nanomaterials in photocatalytic nitrogen fixation is generally ascribed to the additional oxygen vacancies and a large amount of compressive strain which significantly promoted N2 activation and charge separation[62].
Three-dimensional (3D) nanomaterials (assembled superstructures)
-
These nanomaterials have all three dimensions (length, width, and thickness) outside the nanoscale (> 100 nm), but possess nanocrystalline features/structures at the nanoscale[65]. They contain dispersions of 0D, 1D, and 2D nanomaterials in a matrix. One of the advantages of 3D nanomaterials is that they benefit from the combined advantages of the other nanomaterials matrix such as fast carrier separation and transport, shortened diffusion pathways, abundant active and defects sites, and high specific surface area. This results in enhanced structural stability, light utilization, and light absorption. A good example of 3D nanomaterial is tungstic acid/carbon hierarchical (HWO/C) nanoflowers. This photocatalyst was developed for the co-optimization of nitrogen photoreduction[75]. Optimization of the catalyst to a hierarchical structure dramatically increases its photoactivity which is attributed to the chemisorption of nitrogen on carbon. This translates to 58 times (205 μmol·g−1·h−1 NH3) higher increase in NH3 evolution compared to the pure HWO. Therefore, more emphasis should be put on the modification of photocatalysts for efficient N2 fixation.
Synthesis of photocatalytic nitrogen fixing nanomaterials
-
Generally, nanomaterials are synthesized using two approaches i.e., the bottom-up approach and the top-down approach. In the bottom-up approach, nanomaterials are synthesized by assembling the building structure cluster-by-cluster, molecule-by-molecule, or atom-by-atom into a final product using biological or chemical processes. In the top-down approach, a precursor material is reduced in size using chemical or mechanical means such as attrition and milling. Each of the two approaches has its advantages and limitations. In the top-down approach, the major disadvantage is surface structural imperfection, surface defects, impurities, and contaminations. Whereas, the bottom-up approach produces a more homogenous chemical composition material with fewer defects or impurities[65]. Therefore, nanomaterials produced by the bottom-up approach are purer and closer to a thermodynamic equilibrium state. In this review, more emphasis was put only on the methods that produce efficient photocatalysts for nitrogen fixation. This includes sol-gel, ball milling, co-precipitation, hydrothermal/solvothermal, and combustion methods.
Sol-gel synthesis
-
This method is one of the popular and widely used methods in the synthesis of nanomaterials. The method has the advantage of simplicity and yields pure powders with very good morphology, surface area, and size[76]. It involves the reaction of metal salt precursors in their stoichiometric proportions followed by hardening using suitable complexing and chelating agents. The metal salts such as iron nitrates and bismuth nitrates reacted together to form a solution, which is usually homogenous. The solution is then heated at a temperature of 80–90 °C with continuous stirring until a solution is formed. The sol is then dried in an oven at a temperature of 120 °C to form a gel. Finally, the gel is then pre-calcined to give a pure nanomaterial in powdered form. This method was used with success in the synthesis and modification of many N2-fixing photocatalysts[77−80].
Ball milling synthesis
-
This is one of the widely used top-down approaches for the synthesis of nanomaterials. It involves the crushing of suitable powders to reduce their particle sizes and blend the particles into new materials. The typical mills used for these types of processes include attritor mills, planetary mills, vibratory mills, and tumbler ball mills[81]. The general concept of this method is the structural decomposition of grained structures into nanosized particles thereby increasing their surface area and exposing more active sites for reaction. The method was usually used in the modification of nanomaterials[82,83].
Co-precipitation synthesis
-
The co-precipitation method deals with the synthesis of nanomaterials where a solid is precipitated from a solution containing other ions. It is also a simple method of nanomaterials synthesis with a higher yield. It usually results in the production of large grain-size particles with poor homogeneity[80]. The reaction involved the precipitation of metal nitrates through the action of soluble bases such as NH4OH. The precipitate formed is then washed and dried to yield a powdered nanomaterial which can be annealed at different temperatures based on the desired product. Different properties of the nanomaterials such as crystallinity, crystal size, and structure can be controlled using surfactants like sodium dodecyl sulfate, polyvinyl acetate, and cetyltrimethylammonium bromide[84]. Lassoued et al.[85] & Rohokale et al.[86] used this method for the synthesis and enhancement of photocatalytic nanomaterials.
Hydrothermal/solvothermal synthesis
-
This is a solution reaction-based bottom-up approach to nanomaterials synthesis. It has a wide temperature range of synthesis which can happen from room temperature to very high temperatures[87]. Pressure conditions can be manipulated (from high to low) to control the morphology of the materials to be prepared. It usually formed highly dispersed, regular shapes and pure powders with smaller sizes, larger surface areas, and fewer defects[88]. As in the sol-gel method, templating agents can also be used for size control, structure-directing, and crystal growth[89]. Many types of nanomaterials such as RuO2-loaded TiO2, KNbO3, and carbon-doped BiOI have been successfully synthesized through this method[90−92].
Combustion synthesis
-
This is a self-sustaining exothermic reaction method used in nanomaterial synthesis, where metal salts react with organic fuels. It is a rapid, simple, and versatile process, which allows the effective synthesis of various sizes of nanomaterials. The metal salts are mostly metal nitrates, and the organic fuels include urea, hydrazides, and glycine. In the reaction, first, the metal nitrates are dissolved in water followed by the addition of organic fuels with continuous stirring placed on a hot plate until the dissolution process is complete. The mixture is further heated to obtain the nanomaterials after the evolution of gases[93]. This method produces nanomaterials characterized by large particle sizes which can be controlled by the supplement of templating agents[94]. The method is mostly used in the synthesis of oxide nanomaterials[74,79,95].
Advantages and disadvantages of various synthesis methods
Advantages
-
Based on the above synthesis methods, each offers distinct advantages. The sol-gel synthesis method stands out for its simplicity and widespread use. It produces pure powders with excellent morphology, surface area, and size, often resulting in a homogeneous solution that leads to high-quality nanoparticles[96]. Ball milling synthesis, a widely adopted top-down approach, effectively reduces particle sizes, and enhances surface area, exposing more active sites for reactions. This method is particularly advantageous for its ability to decompose grain structures into nanosized particles.
Co-precipitation synthesis is another simple and high-yield method. It allows for the control of nanomaterial properties such as crystallinity and crystal size using surfactants, making it versatile for producing tailored nanoparticles[84]. Hydrothermal and solvothermal synthesis methods offer flexibility with a wide temperature range and controllable pressure conditions, resulting in highly dispersed, regular-shaped, and pure powders with larger surface areas and fewer defects[87]. The ability to manipulate these conditions makes these methods ideal for creating well-defined nanomaterials. Combustion synthesis, known for its rapid and straightforward nature, is highly versatile and effective for producing various sizes of nanomaterials. Utilizing a self-sustaining exothermic reaction, this method is efficient for synthesizing oxide nanomaterials quickly.
Disadvantages
-
Despite their advantages, each synthesis method for carbon-based nanoparticles also has its limitations. The sol-gel method, while simple, requires precise temperature control and continuous stirring. Additionally, the process involves an extra pre-calcination step to obtain the final product, which can be time-consuming. Ball milling synthesis, although effective in reducing particle sizes, can introduce surface defects and structural imperfections[97]. The milling process is also prone to impurities and contaminations, which can affect the quality of the final product.
The co-precipitation method, while simple and high-yield, often results in large grain-size particles with poor homogeneity. Thorough washing and drying are required to obtain the final product, adding to the complexity of the process[98]. Hydrothermal and solvothermal synthesis methods, despite their flexibility, can be complex due to the need for controlled temperature and pressure conditions. These methods may also require the use of templating agents for size and structure control, adding another layer of complexity to the process. Combustion synthesis, while rapid and simple, often produces larger particle sizes, which can be controlled with templating agents but still presents a challenge[99]. Moreover, this method is mostly suitable for synthesizing oxide nanomaterials, limiting its versatility for other types of nanoparticles.
-
Band gap energy can be described as the distance of electron travels between the valence band and the conduction band. It is the minimum amount of energy that an electron required to be excited from the valence band up to the conduction (free of its bound state) where it can participate in a reaction. The band gap energy is the key determinant of the photocatalytic performance of a nanostructured photocatalyst. For a material to catalyze with efficient photocatalytic properties, the band gap energy must not be smaller than 3 eV. This is to allow the catalyst to utilize the visible region (42% to 43%) of the solar energy. While 3 eV is the maximum band gap for efficient photocatalysis, the minimum band gap should not be less than 1.23 eV. This is because water splitting cannot occur if a photocatalyst has a band gap of less than 1.23 eV[100]. Table 1 gives some photocatalysts with their band gap energies. An effective photocatalyst mostly possesses bandgaps greater than 2 eV. Hence, it is imperative to target a material that has a band gap of 2–3 eV for effective and efficient photocatalytic nitrogen fixation.
Table 1. Photocatalyst for photocatalytic nitrogen fixation.
Photocatalyst Band gap
energy (eV)Yield (NH3) Ref. Bi2O2CO3 2.65 1,175.78 μmol·L−1·g−1·h−1 Feng et al.[101] I-g-C3H4 2.68 200.8 mg·L−1·g−1 Hu et al.[102] La2TiO5 4.07 158.13 μmol·g−1·h−1 Song et al.[103] C-BiOI 1.81 311 μmol·g−1·h−1 Zeng et al.[92] g-C3H4 2.74 150 mg·L−1·h−1·g−1 Hu et al.[104] FeS2/Fe-Pal 1.30 147 μmol·g−1·h−1 Ye et al.[105] In2O3/In2S3 2.77 40.04 μmol·g−1·h−1 Xu et al.[106] Ag/B-g-C3H4 2.82 5.19 mg·h−1·g−1 Yao et al.[107] N-g-C3H4 2.60 531.24 μmol·L−1·g−1·h−1 Liu et al.[108] Bi2S3 1.48 51.04 μmol·g−1·h−1 Lan et al.[109] Bi2S3@PCN-2 1.61 3,880 μg·h−1·g−1 Chen et al.[110] W18O49/g-C3N4 2.83 64.8 μmol·gcat−1·h−1 Huang et al.[111] TCN/ZnS/ZnIn2S4 2.59 136.56 μmol·L−1 Sun et al.[112] Redox potential of a photocatalyst
-
The redox potential of a photocatalyst gauges its inclination to engage in reduction or oxidation reactions, involving the acceptance or donation of electrons, respectively. In the realm of photocatalytic processes, the efficacy of redox reactions hinges significantly on two key factors: the reduction potential of nitrogen molecules[113] and the energy band positioning within the photocatalyst[114]. For successful photocatalytic nitrogen fixation, a photocatalyst must possess a conduction band situated above the reduction potential required for N2 hydrogenation (0.55 V), while concurrently, its valence band should be positioned below the oxygen evolution potential (0.81 V)[113]. This highlights the pivotal role played by the bandgap energy when selecting a suitable material for photocatalytic nitrogen fixation.
Kinetic limitations
N2 adsorption and activation
-
The critical and rate-controlling step in the fixation of N2 lies in the process of adsorption and activation of nitrogen molecule. N2 molecules, known for their chemical stability and low coordination, present a formidable challenge in terms of surface adsorption on a catalyst. Delving into the electronic configuration of nitrogen atoms (as depicted in Fig. 2), five valence electrons residing in the 2s and 2p orbitals are found, with three of them remaining unpaired. Upon bonding, these atomic orbitals undergo hybridization, giving rise to four bonding orbitals housing two σ orbitals and two π orbitals, along with four anti-bonding orbitals comprising two σ* orbitals and two π* orbitals[48]. This delineates a substantial energy gap of 10.82 eV between the highest occupied molecular orbital and the lowest unoccupied molecular orbital, leading to a high ionization energy of 15.58 eV[115]. Consequently, the N≡N triple bond exhibits remarkable stability, demanding the input of a substantial 945 kJ·mol−1 of energy for cleavage to occur[49]. It is this robust bond energy that imposes a formidable barrier to the adsorption and activation of N2 in the fixation process.
Drawing inspiration from the realm of biological nitrogen fixation, which finds its muse in natural nitrogenase, photocatalytic nitrogen fixation has witnessed the development of photocatalysts employing various transition metals (such as Fe, Mo, V, Bi) for the chemical fixation of atmospheric N2. This achievement hinges upon the potent interaction between these metals and the 2p orbital of nitrogen, enabling N2 to become chemically adsorbed on the catalyst's surface. This interaction, in turn, forges a pathway for the transfer of electrons from the catalyst to N2, thereby facilitating N2 activation. These donated electrons find their way into the anti-bonding π* orbitals of N2, causing an elongation of the bond length and a corresponding reduction in bond energy, ultimately leading to the cleavage of the N≡N bond. This intricate dance of electrons culminates in the adsorption and activation of N2. Consequently, the selection of a catalyst with a pronounced affinity for coordinating with nitrogen assumes paramount importance in the pursuit of efficient N2 fixation.
Electrons and hole separation and mitigation of charge recombination
-
In the realm of photocatalytic processes, a significant energy gap between the brimming valence band (VB) and the unoccupied conduction band (CB) dictates that electrons can migrate only when furnished with ample energy. When light energy permeates a nanomaterial, an electron can absorb a photon of equivalent or higher energy than the material's band gap energy. This absorption elevates an electron from the VB to the CB, concurrently generating a vacancy or 'hole' in the VB. This pivotal mechanism, driven by light, underpins the separation of electrons and holes, a prerequisite step in the photocatalytic reaction cascade. These photogenerated entities subsequently traverse to the nanomaterial's surface, where they instigate reduction reactions with adsorbed nitrogen molecules. One of the most formidable challenges confronting many photocatalytic reactions is the propensity for photogenerated electrons and holes to recombine, dissipating energy and thereby diminishing reaction rates. Consequently, this recombination phenomenon erodes photocatalytic efficiency[116,117]. Hence, a pressing need arises to stymie recombination and bolster photocatalytic reaction efficiency.
Recent endeavors have seen a surge in studies aimed at extending the lifespan of photogenerated carriers, thus mitigating recombination and enhancing reaction efficiency. These strategies encompass the use of co-catalysts[118,119], modifications involving functional groups[120], surface engineering[103], the formation of heterojunctions[121,122], and doping techniques[103]. A comprehensive exploration of these methods will follow in the subsequent section.
-
A co-catalyst, a supplementary material introduced alongside a well-established catalyst serves the purpose of augmenting the overall catalytic performance. The role of a co-catalyst in enhancing photocatalysis encompasses various facets, including the reduction of activation energy, bolstering the stability of photocatalytic materials, impeding the undesirable recombination of photogenerated charges, and facilitating the separation of electrons and holes. The incorporation of a co-catalyst into a photocatalyst, such as CdS, has proven highly effective in expediting the separation of charges within the photocatalyst. This, in turn, substantially amplifies the photocatalytic capabilities of the material for nitrogen fixation[119]. According to insights from Liu et al.[108] and Hayat et al.[123], the introduction of a co-catalyst bestows three pivotal advantages that measurably enhance photocatalytic performance: firstly, an augmentation in light absorption capacity, thereby broadening the catalyst's utilization of incident light; secondly, an improvement in the transfer of photogenerated electrons and a reduction in carrier recombination rates; and lastly, an expansion of active sites for photocatalytic nitrogen fixation activities.
Apart from putting more emphasis on the N2 reduction reaction, the water oxidation reaction as the other critical reaction of the photocatalytic nitrogen fixation was enhanced using a cocatalyst. Mohebinia et al.[124] enhance oxygen evolution reaction through the application of cobalt oxyhydroxide as a cocatalyst to photocatalyst bismuth oxychloride. This resulted in a 4.6-fold improvement in the ammonia production rate compared to normal bismuth oxychloride. Therefore, a cocatalyst application points to an applicable and promising strategy for improved photocatalytic nitrogen fixation studies.
Creation of oxygen, sulphur, and nitrogen vacancies
-
Vacancy defects, including nitrogen vacancies (NV), oxygen vacancies (OV), and sulfur vacancies (SV), constitute pivotal factors in bolstering the catalytic efficacy of photocatalysts engaged in nitrogen fixation[125]. These vacancies house a surplus of localized electrons, which they readily contribute to N2 molecules, fostering the formation of chemical bonds between neighboring atoms and N2. This transformative process activates the otherwise inert N2 molecule. Beyond N2 activation, vacancies play a dual role by markedly enhancing the separation of charge carriers through the entrapment of photoexcited electrons and facilitating interfacial charge transfer. Furthermore, vacancies serve as active sites for the adsorption and activation of reactant molecules. Intriguingly, vacancies exert an influence on the band gap energy, narrowing it and consequently expanding the photocatalyst's capacity to absorb light[126].
It is essential to recognize that not all vacancy defects yield enhanced catalyst performance. According to Yue[127], bulk defects impede the migration of photogenerated electrons and suppress the separation of electron-hole pairs. In contrast, only surface defects enhance and support the photocatalytic performance of nitrogen fixation. In catalysts with narrower band gaps, the introduction of defects can reduce the band gap energy, potentially diminishing the oxidation and reduction capabilities of the photocatalyst[126]. This, in turn, impacts water-splitting reactions, which necessitate a band gap greater than 1.23 eV[100]. Consequently, it becomes imperative to target photocatalysts featuring a substantial band gap when considering the introduction of vacancy defects.
Use of doping techniques
-
Doping is the act of introducing a foreign atom into the lattice of a known photocatalyst to induce defect states in the chemical and electronic properties of the catalyst. In photocatalytic nitrogen fixation, the dopant sites played a key role in the adsorption and activation of N2, while also promoting the separation of charge carriers[128]. Transition metals are the most considered dopants due to their unfilled d orbitals[129]. The unfilled d orbitals can donate electrons to N2 molecules through its antibonding π orbitals. This weakens and polarizes the N≡N triple bond leading to its cleavage. A good example of the most studied transition metal dopant for nitrogen activation in photocatalytic nitrogen fixation is iron[75,79,130,131]. Observation shows that the N≡N bond is stretched from 1,157 to 1,181 Å as N2 molecules adsorb onto the Fe3+ sites[132]. This confirmed the direct role of Fe in the cleavage of the triple bond and activation of the N2 molecule, thereby enhancing the NH4+ production rate. In addition, doping causes charge imbalance in the crystalline phase of some photocatalysts resulting in oxygen defects and vacancies[130]. Also, photocatalytic dopants have the potential to act as a trapping center and inhibit the recombination of photogenerated charge.
Formation of heterojunctions
-
The concept underpinning the creation of a heterojunction involves the fusion of two dissimilar photocatalysts characterized by unequal band structures. This fusion serves to align their respective band structures, expanding the range of light absorption across a broader spectrum[133]. Through this approach, a significantly enhanced photocatalytic performance is achieved, stemming from augmented light absorption and improved separation of photogenerated electrons and holes, thereby enhancing overall photocatalytic efficiency. Heterojunctions can be forged between catalysts of varying dimensions, giving rise to a distinctive geometric arrangement that optimizes the combined properties and synergistic effects between the catalysts[134].
The outcomes of heterojunction catalysis have been nothing short of remarkable, with the resulting hybrids frequently exhibiting exceptional visible light absorption, thereby enhancing light-driven photocatalytic N2 photofixation[135−137]. In scenarios where one photocatalyst possesses conduction and valence bands higher in energy than the other, it leads to the migration and confinement of photogenerated holes and electrons in opposite directions[138]. This effect further extends the overall span of the conduction and valence bands, consequently facilitating the increased involvement of photogenerated electrons and holes in photocatalytic reactions. A study by Tu et al.[139] demonstrated that the unique interface structure in a TiO2/CdS heterojunction significantly influenced carrier separation and migration, achieving a photocatalytic nitrogen fixation rate of 57.4 μmol·g−1·h−1. This improvement was attributed to the efficient charge separation and prolonged lifetime of charge carriers due to the well-engineered interface, highlighting the critical role of specific structural and chemical bonding at the interface in enhancing photocatalytic performance.
-
This comprehensive review offers an insightful examination of current knowledge pertaining to the reaction mechanisms and photocatalysts employed in Photocatalytic Nitrogen Fixation (PNF). It's noteworthy that, among the four approaches to natural and artificial nitrogen fixation, PNF stands out as the most environmentally friendly and sustainable method for ammonia and nitrate synthesis. A profound understanding of PNF mechanisms hold paramount importance in the quest to identify and design efficient photocatalytic systems. Additionally, developing new characterization techniques to investigate the dynamic processes during PNF reactions in real-time could provide further insights into the reaction pathways and intermediates, facilitating the design of more effective photocatalysts.
Moreover, there exists a compelling need to delve deeper into the exploration of materials possessing favorable attributes such as suitable band gaps, numerous active sites, optimal defect densities, and expansive specific surface areas. These attributes are pivotal for enhancing the adsorption and activation of N2 molecules. Addressing the thermodynamic and kinetic constraints of PNF necessitates the development of highly efficient photocatalytic systems. This can be achieved through methodologies like morphology engineering, doping techniques, the judicious modification of cocatalysts, the creation of vacancies, and the establishment of heterojunctions. Further interdisciplinary collaboration among chemists, material scientists and engineers will be essential to overcome these challenges and translate laboratory-scale successes into industrial applications.
In a promising light, the ongoing exploration and advancement of efficient photocatalysts and techniques for PNF utilizing sunlight under ambient conditions holds tremendous potential for ammonia production while concurrently curbing carbon dioxide emissions. It's conceivable that photocatalytic fixation may usher in a transformative shift, potentially complimenting the Haber-Bosch process with more sustainable and widely accessible methods in the future. Continued investment in research and development, coupled with supportive policies and incentives will be crucial in accelerating the transition towards these greener technologies and achieving broader environmental and economic benefits.
-
The authors confirm contribution to the paper as follows: All of the four authors contributed equally to the development and actualization of this work.
-
Data sharing not applicable to this article as no datasets were generated or analyzed during the current study.
The authors express their deep gratitude to Ahmadu Bello University (ABU), located in Zaria, Nigeria. Their appreciation also extends to the Tertiary Education Trust Fund (TETFUND), Nigeria, for providing the necessary funding for this research project through the Institution Based Research (IBR) Fund. The grant, identified by the reference number TETF/DR & D/UNI/ZARIA/IBR/2020/VOL.1/1, has played a pivotal role in facilitating this study.
-
The authors declare that they have no conflict of interest.
-
# Authors contributed equally: Sharhabil Musa Yahaya, Nafiu Abdu, Ibrahim Abubakar Aliyu, Bello Mukhtar
- Copyright: © 2024 by the author(s). Published by Maximum Academic Press, Fayetteville, GA. This article is an open access article distributed under Creative Commons Attribution License (CC BY 4.0), visit https://creativecommons.org/licenses/by/4.0/.
-
About this article
Cite this article
Yahaya SM, Abdu N, Aliyu IA, Mukhtar B. 2024. Revolutionizing nitrogen fixation: communicating the potentials of nanostructured photocatalysts for sustainable ammonia/nitrate synthesis. Circular Agricultural Systems 4: e015 doi: 10.48130/cas-0024-0014
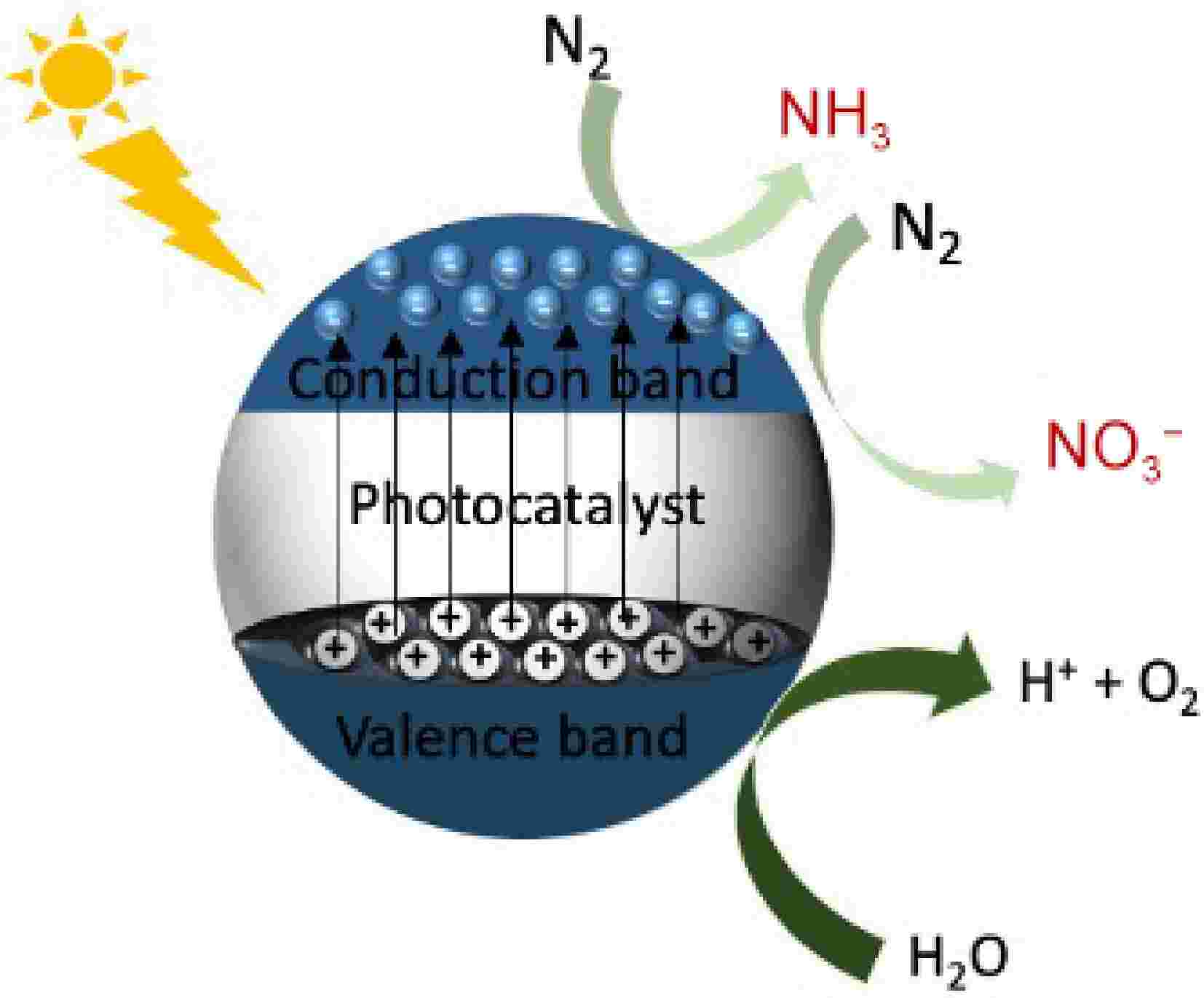