-
In higher plants, haploid microspores are formed through meiosis in the anther and typically develop into mature pollen for pollination. However, under specific in vitro stress conditions, these microspores can be reprogrammed toward embryogenesis, leading to the formation of embryo. Following induction, a subset of microspores, known as responsive microspores, acquire totipotency and embryogenic competence, while others do not survive[1,2]. This process, known as microspore embryogenesis (or androgenesis), is particularly valuable in plant breeding, as it enables the production of double haploid (DH) plants, which are fully homozygous within a single generation[3].
Microspore embryogenesis induced by stress also provides a valuable model for studying cell reprogramming and the transition from differentiation to proliferation[4]. However, the efficiency of this process is constrained by various factors, leading to bottlenecks at specific developmental stages. Despite its usefulness in breeding programs, the in vitro system often exhibits low efficiency, particularly in many crops[5]. Further understanding of the induction processes could help identify new targets and develop strategies to enhance the efficiency of in vitro embryogenesis, even in species that are typically resistant to this method.
Progress in understanding the regulatory mechanisms of microspore embryogenesis induction has been limited by challenges in studying the early stages using biochemical and molecular techniques, as well as the difficulty of applying genetic approaches to embryogenesis-responsive genotypes. These techniques are crucial for localizing key molecules and identifying differential gene expression in embryogenic structures from the initial stages, distinguishing them from non-embryogenic ones through advanced imaging microscopy technologies[6,7]. Recent advances have identified several cellular processes involved in regulating stress-induced microspore embryogenesis, although their exact roles remain incompletely understood.
This review highlights the key mechanisms influencing the induction of microspore embryogenesis, with a focus on cytoskeleton dynamics, cell wall remodeling, programmed cell death (PCD), autophagy, hormones, and chromatin modifications. In addition, current cell and molecular biology techniques offer new strategies to enhance the efficiency of microspore embryogenesis through pharmacological treatments with small molecules that modulate these processes[8−10]. These advancements not only deepen our understanding of the mechanisms underlying microspore embryogenesis but also hold significant potential for increasing haploid plant production in crop breeding.
-
Embryo-forming microspore typically exhibit a round shape with a centrally located nucleus and vacuoles[11]. During the gametophyte pathway, mid-late uninucleate microspores contain a large vacuole that occupies most of the cell’s internal space, positioning the nucleus at one side of the spore wall. However, upon exposure to elevated temperatures, microspores transition to an embryogenic state, marked by significant volume expansion. This transition includes the elimination of starch grains, the breakdown of the large central vacuole, the relocation of the nucleus to the center, and a reduction in cytoplasmic volume, resulting in a radial expansion that forms a star-like structure (SLS)[12]. The appearance of this SLS is the first visible sign of microspore embryogenesis[13]. However, this structure is not always a reliable marker of embryogenesis initiation, as it can also appear in cultured microspores that fail to develop into embryos[14,15]. Therefore, identifying a dependable molecular marker that accurately indicates the switch in microspore cell fate toward androgenesis is crucial. Such a marker would provide a valuable tool for studying the molecular mechanisms of androgenesis initiation and could significantly enhance the efficiency of DH induction.
The microtubule (MT) cytoskeleton plays a key role in responding to stress due to its dynamic nature, which allows it to reorganize in response to environmental and developmental stimuli[16]. Cytoskeletal changes are essential for regulating processes such as cell division, polarity, cell wall formation, intracellular transport, and autophagy[16]. During the stress induction phase of microspore embryogenesis, key events such as microspore enlargement, nucleus migration to the center (forming the SLS), and preprophase band formation before symmetric division all rely on the organization and dynamics of cortical microtubules (CMT) and endoplasmic microtubules (EMT)[17]. In embryogenic cells, the MT cytoskeleton loses its polarity, and microtubules undergo extensive reorganization, likely playing a central role in the newly induced symmetric division[18].
The involvement of the cytoskeleton in microspore embryogenesis induction has sparked interest in the effects of tubulin-targeting agents such as colchicine, cytochalasin D, and n-butanol[19−21]. In wheat (Triticum aestivum), stress treatment with mannitol, followed by the application of n-butanol, a known microtubule-disrupting agent, has been shown to enhance microspore embryogenesis and plant regeneration[22]. In uni-nucleated, mannitol-treated microspores, severe fragmentation of CMT and EMT is observed, although a network of short EMT bundles persists to protect the nucleus. Subsequent treatment with n-butanol leads to further depolymerization of both CMT and EMT, accompanied by the formation of MT aggregates in the perinuclear region[22]. It is possible that these MT bundles facilitate the migration of the nucleus to a central position prior to division. Although further research is needed to fully understand the mechanisms underlying these MT modifications, these studies suggest that targeted treatments affecting MT dynamics could offer new strategies for inducing microspore embryogenesis in species with low responsiveness.
-
Plant cell walls are complex structures composed of polysaccharides, proteins, and aromatic compounds[23]. Beyond their role in maintaining cell shape, cell walls are integral to plant growth, cell differentiation, intercellular communication, water transport, and defense mechanisms[24]. In viable embryos, the cell wall profile is distinct, characterized by thin walls rich in arabinogalactan proteins (AGPs), both highly and low methyl-esterified pectin, and callose. These embryos also feature a subintinal layer with a notably high concentration of callose, which, while not necessarily thick, is crucial for the embryo's structural integrity and function[25]. Pectin, a major component of plant cell walls, is typically found in a highly methylated form[26]. The degree of pectin methylation is regulated by pectin methylesterases (PMEs)[26], which modify the rigidity and flexibility of the cell wall[27]. Studies utilizing monoclonal antibodies and Yariv reagents[28] have shown that AGPs play a role in processes such as cell division, programmed cell death, pollen tube differentiation, and embryonic pattern formation[29].
Cell wall remodeling is critical during microspore embryogenesis[30]. In Brassica napus, differential expression of pectin PMEs has been observed during this process. Non-esterified pectin is associated with the gametophytic pathway, while esterified pectin signals totipotency and the onset of microspore embryogenesis[31]. This indicates that PME-mediated modifications to the pectin structure are pivotal for microspore embryogenesis, facilitating cell wall remodeling. Auxin, a hormone linked to organogenesis in Arabidopsis thaliana, requires pectin demethylation to reduce cell wall stiffness and promote its function[5]. When microspores were treated with indole-3-acetic acid (IAA), almost all PME genes were upregulated, leading to accelerated pectin decomposition[32]. This results in a significant reduction in pectin content, the degradation of pollen mother cell walls, and faster release of tetrad microspores[32], suggesting that cell wall deterioration may promote microspore advancement.
Except for pectin esterification, AGP also plays a critical role in microspore embryogenesis. The incorporation of arabinogalactans, an AGP source, in T. aestivum microspore cultivation has been shown to enhance the likelihood of embryogenesis[33]. However, treatments such as heat shock combined with the histone deacetylase inhibitor trichostatin A (TSA) could induce cell walls that are unfavorable for embryogenesis progression, featuring reduced AGP levels, weakened inner wall adhesion, and altered pectin composition[34]. This underscores the importance of both PMEs and AGPs in cell wall remodeling during microspore embryogenesis, as they influence cell rigidity and embryonic development. A recent study also suggests that cell wall lignification may also be necessary for somatic embryogenesis in Areca catechu[35], suggesting that other cell wall components might impact microspore embryogenesis. This is an area that remains largely unexplored.
-
PCD is a gene-regulated process essential for the development and homeostasis of multicellular organisms[36,37]. This mechanism is universally observed, from single-celled prokaryotes to complex eukaryotes[38]. In the context of microspore embryogenesis, PCD plays a crucial role, particularly under stress conditions such as heat shock, starvation, cold, ethanol, and gamma irradiation, which lead to the death of numerous microspore-containing anthers[39].
Microspore embryogenesis involves two key stages: the formation of multicellular structures (MCS) within the isolated microspore's outer wall and the differentiation of these MCS into embryo-like structures (ELS). PCD occurs during the transition from MCS to EL[13]. For instance, under mannitol stimulation, MCS differentiate into two types of cells: vegetative cells and germ cells. While germ cells undergo PCD and die, vegetative cells continue to develop into ELS, eventually forming globular embryos[12]. In barley (Hordeum vulgare), PCD in vegetative cells often preceded the rupture of the outer wall, facilitating the release and development of globular embryos by removing the outer wall[12].
Stress conditions also induce an increase of reactive oxygen species (ROS)[40], which are closely associated with autophagy[41]. In H. vulgare microspores, stress treatments lead to elevated ROS levels, which triggered the formation of autophagosomes — structures that contain phagocytic substances and organelles. These autophagosomes activate hydrolytic proteases, contributing to cellular degradation. The application of specific inhibitors has been shown to reduce microspore death and improve rates of microsporogenesis[42]. Effective microspore embryogenesis in H. vulgare has been linked to the accumulation of ascorbate and the high activity of enzymes that regulate its redox status. The most successful treatments involve low temperatures and the exogenous application of mannitol, with or without reduced glutathione[43]. By adjusting culture conditions, including antioxidant levels and nutrient content, researchers can more effectively manage ROS levels, enhancing microspore viability and promoting embryogenesis[44]. Inhibitors of autophagy and cysteine proteases have been shown to reduce cell death, thereby promoting embryogenesis[10].
While autophagy is closely linked to cell death, it plays a dual role in plants. Autophagy is not only a mechanism for cellular degradation and recycling but is also involved in various essential developmental processes[45,46]. In addition to its role in inducing PCD, autophagy influences cell fate determination[47]. A recent study showed that autophagy plays a role in promoting tobacco (Nicotiana tabacum) microspore cell fate transition, which might be involved in lignin biosynthesis and chromatin decondensation for promoting reprogramming for androgenesis initiation[48].
-
The role of plant growth regulators (PGRs) is a key focus in microspore embryogenesis research. These critical signaling molecules regulate plant growth and development and initiate signal transduction pathways in response to various environmental stimuli[49]. Among PGRs, auxin and cytokinins (CKs) play significant roles, acting synergistically or antagonistically depending on context and relative concentrations[50].
Auxins, the first plant hormones identified, is essential for cell growth, division, and embryogenesis[51,52]. IAA, the first auxin discovered, is still the most widely utilized for inducing microspore embryogenesis[53]. More stable synthetic auxin analogs, such as 2,4-dichlorophenoxyacetic acid (2,4-D), are often used as culture media supplements for both dicotyledonous and monocotyledonous plants[54]. Interestingly, several inhibitors of auxin biosynthesis (7-azaindole, AZI) or auxin polar transport, such as 2,3,5-triiodobenzoic acid (TIBA), 1-N-naphthylphthalamic acid (NPA), and 1-naphthoxyacetic acid (1-NOA), along with auxin analogues α-(o-chlorophenoxy)-isobutyric acid (OCPIB) and p-Chlorophenoxyisobutyric acid (PCIB), have also been frequently employed for microspore embryogenesis. These compounds disrupt auxin homeostasis and thereby influence embryo development[55]. However, the results can be ambiguous or contradictory. For instance, the inhibitors such as AZI[56], OCPIB[56], TIBA[57], and PCIB[58] have been reported to enhance embryo formation. In contrast, NPA[59] and 1-NOA[55], both potent synthetic auxin inhibitors, have been associated with a negative effect on microspore embryogenesis.
To date, most studies have focused on exogenous auxin in culture media, with only a few studies on the effects of endogenous auxin on microspore embryogenesis. Previous studies have shown that endogenous auxins accumulated in early microspore embryo cells of B. napus and Quercus suber[60,61]. In the microspore-derived embryos of B. napus, auxin has been monitored by using the DR5 and DR5rev reporter gene systems at cellular resolution[62]. In addition, the involvement of endogenous auxin in microspore reprogramming and in vitro embryo formation has been further revealed, with de novo biosynthesis of endogenous auxin and early accumulation of IAA in proembryo detectable from the first embryogenic divisions[61]. Moreover, the expression of both tryptophan aminotransferase of Arabidopsis 1 (BnTAA1) and Peptidyl-prolyl cis-trans isomerase Pin1 (BnPIN1) is upregulated throughout the initiation and progression of microspore embryogenesis, correlating with increased IAA levels[61]. Altogether, these findings clearly indicate that auxin biosynthesis, activity, and transport are essential for stress-induced microspore embryogenesis.
CKs, along with auxin, are crucial regulators of plant growth and development[63]. The balance of these two hormones, which usually act antagonistically, is vital for cell division and differentiation. Exogenous CKs have been tested in various anther culture systems, yielding variable results[64]. However, the role of endogenous CKs in microspore embryogenesis remains unclear. A recent study demonstrated that effective embryogenic development of isolated triticale (× Triticosecale Wittm.) microspores requires high concentrations of auxins and moderate levels of CKs, with active trans isomers released by co-cultured ovaries playing a critical role. Notably, high induction efficiency is associated with a significantly lower ratio of active auxin to active CKs[65].
Abscisic acid (ABA), a known ubiquitous plant stress hormone, plays a role in the strees-signal transduction in inducing microspore embryogenesis[66]. ABA levels of microspores increased in microspore embryogenesis induction in Hordeum vulgare[67] and T. aestivum[68], suggesting the positive influence of ABA accumulation on effectiveness. This effect also has been confirmed by treatments with exogenous ABA or its inhibitor fluridone[68,69]. In contrast, higher levels of endogenous ABA have been shown to significantly reduce regeneration efficiency. Nevertheless, the role of ABA in microspore reprogramming is still complex. On the one hand, it acts as an anti-stress factor that enhances microspore viability during microspore embryogenesis induction. On the other hand, ABA-induced signaling cascades activate various genes, primarily those controlling the synthesis of late embryogenesis abundant (LEA) proteins. In addition, ABA also influences the activity of enzymes, modulates the redox status, and interacts with other PGRs[70−72]. Therefore, a specific homeostasis of PGRs and the crosstalk between auxin, CKs, and ABA seems to be more critical for effective microspore embryogenesis than the absolute levels of individual PGRs[71].
Despite extensive research of phytohormone on microspore culture, the effects of other phytohormones, such as brassinosteroids (BRs), gibberellins (GAs), jasmonic acid (JA), salicylic acid (SA), and ethylene on microspore embryogenesis, are still underexplored. A recent study introduced a novel strategy to enhance microspore embryogenesis in B. napus and H. vulgare using synthetic small molecule inhibitors of mammalian glycogen synthase kinase 3β (GSK-3β)[73]. Plant GSK-3-like plays a key role in hormonal signaling networks during development and stress responses[74]. The inhibitor thiadiazolidinone-8 (TDZD-8) was found to suppress GSK-3 activity in microspore cultures, leading to increased expression of embryogenesis-related genes such as FUSCA3 (FUS3), LEAFY COTYLEDON2 (LEC2), and AGAMOUS-LIKE15 (AGL15)[73]. This suggests that GSK-3 kinases, particularly the BR-INSENSITIVE 2 (BIN2) regulator of BR signaling[32,75,76], are involved in microspore embryogenesis. During this process, genes associated with BR biosynthesis and signaling, including CONSTITUTIVE PHOTOMORPHOGENESIS AND DWARFISM (CPD), GSK-3/BIN2, BRI1-EMS-SUPPRESSOR1 (BES1), and BRASSINAZOLE-RESISTANT1 (BZR1), are upregulated, while the BR catabolic gene PHYB ACTIVATION-TAGGED SUPPRESSOR1 (BAS1) is repressed, indicating activation of BR pathway[73]. TDZD-8's ability to enhance BR signaling components suggests that it may mimic the effect of BR, highlighting the crucial role of BR in microspore embryogenesis[73].
GA is involved in a wide range of developmental responses in plants[77]. In microspore cultures of B. napus and Solanum tuberosum, GA3 has been shown to enhance plantlet regeneration, primarily by promoting embryo axis elongation and accelerating maturation[78]. Similarly, Ahmadi et al. reported that GA3 treatment resulted in the highest percentage of B. napus plantlet regeneration[79]. The application of the GA-biosynthesis inhibitor uniconazole in significantly inhibited axis elongation of globular embryos in B. napus[80].
JA regulates stamen development and fertility under basal conditions, affect root growth and trichome formation under stress conditions, and control defense responses against insect herbivores and pathogens[81]. In H. vulgare anther cultures, microspore embryogenesis-induction treatment resulted in higher expression of three genes encoding enzymes involved in JA biosynthesis[82]. Ahmadi et al.[72] claimed that the supplementation of induction medium with JA for 24 h improved embryo yield in microspore cultures of B. napus. Moreover, the addition of JA for 12 h resulted in better plantlet regeneration.
SA plays a role in the defense mechanisms against biotic and abiotic stress[83]. Several studies have explored the application of SA to culture media to improve microspore embryogenesis efficiency. Ahmadi et al.[72] reported a positive effect of application of SA on the yield of microspore-derived embryos in B. napus. The mechanism of SA action may be linked to its ability to increase the activity of superoxide dismutase (H2O2-producing enzyme) while inhibiting the activities of ascorbate peroxidase and catalase (H2O2-decomposing enzymes). This inhibition leads to endogenous H2O2 accumulation, which is thought to initiate microspore embryogenesis[70].
Ethylene is a gaseous plant hormone involved in various developmental processes[84], and it plays a role in in vitro callus growth, organogenesis, and embryogenesis[85]. Embryogenesis in H. vulgare can be stimulated by both promoters and antagonists of ethylene, depending on the genotype[86]. It suggests that the response is influenced by the amount of ethylene produced, with an optimal concentration required for the initiation of microspore embryogenesis. Positive effects have often been observed with substances known as inhibitors of ethylene action, such as silver nitrate[87], activated charcoal[88], aminoethoxyvinylglycine (AVG), and cobalt chloride[89]. Conversely, it reported benefits from ethylene precursors such as 1-aminocyclopropane-1-carboxylic acid (ACC) and promoter Ethephon (ETP), which enhance microspore embryogenesis initiation in the anther cultures of H. vulgare[90] and Avena sativa[91].
Overall, both endogenous and exogenous hormonal regulation are crucial for effective microspore embryogenesis induction. However, most systems do not require exogenous hormones as inducers; instead, transient physical (thermal) or chemical stress is essential for triggering transition in developmental cell fate, likely as a response mediated by endogenous hormones. Therefore, the precise functions and regulatory networks of various endogenous hormones in microspore embryogenesis remain largely unknown, presenting significant opportunities for future research.
-
Emerging studies underscore the increasing recognition of epigenetic processes as pivotal regulators of gene expression and genomic stability during adaptive responses to developmental changes[91]. These epigenetic modifications are particularly significant in reproductive development, responses to abiotic stress, and plant regeneration—all essential to microspore embryogenesis[92−94]. The primary epigenetic mechanisms in plants include DNA methylation, histone post-translational modifications (PTMs), and the regulation of small RNA and long noncoding RNA pathways[95].
DNA methylation is a stable and heritable modification that can change in response to developmental and environmental factors[96]. During microspore embryogenesis, typically conducted under stress conditions, a reduction in DNA methylation has been observed[97,98]. The change in the developmental program and the initiation of embryogenesis influence the functional organization of the nuclear domains, including chromatin condensation state[48]. Open chromatin typically enhances the accessibility of the genome to the transcription machinery, whereas closed chromatin represses gene expression by restricting this accessibility[99]. Quantitative biochemical assays and immunolocalization of 5-methyl-deoxy-cytosine (5mdC) demonstrated that microspore reprogramming and the initiation of embryogenesis involved global DNA hypomethylation. Following induction, early microspore-induced proembryos exhibited a decondensed chromatin pattern characterized by low DNA methylation, as revealed by 5mdC labeling[97]. In B. napus microspore embryogenesis, microspores cultured at 32 °C exhibited DNA hypomethylation[98]. In winter triticale (× T. Wittm.), treatment with DNA methylation inhibitors such as 5-azacytidine (AzaC) and 2’-deoxy-5-azacytidine (DAC) resulted in decreased DNA methylation and increase microspore embryogenesis, suggesting that hypomethylation may enhance the induction of embryogenesis[100]. In contrast, further embryo development is characterized by a progressive increase in global methylation, accompanied by heterochromatization associated with cellular differentiation. In B. napus, the expression of the DNA methyltransferase gene MET1 is upregulated during microspore embryogenesis[101]. When 5-azacytidine (AzaC) is applied at early stages, it induced DNA hypomethylation and promoted the initiation of microspore embryogenesis in both B. napus and H. vulgare[8]. The combination of AzaC and low temperature as an inducing treatment enhanced the expression of genes such as GLUTATHIONE S-TRANSFERASE (GSTF2), TAPETUM DETERMINANT1 (TPD1-like), and SOMATIC EMBRYOGENESIS RECEPTOR KINASE 2 (SERK2), which are involved in regulating microspore embryogenesis[102]. In cabbage (Brassica oleracea), differentially expressed genes targeted by DNA methylation and miRNAs during heat shock were primarily linked to ROS metabolism and ABA signaling, indicating that DNA methylation and miRNA (microRNA) regulation may influence microspore embryogenesis by modulating these pathways[103]. Recent findings further support the idea that DNA hypomethylation is critical for regulating chromatin remodeling and switching the gene expression program during the induction of microspore embryogenesis.
Histone-tail PTMs, such as acetylation and methylation, directly or indirectly influence the interaction between histones and DNA, thereby affecting transcriptional regulation[95]. Acetylation is typically associated with transcriptional activation, while methylation is linked to repression. Chromatin-modifying enzymes, including histone lysine methyltransferases (HKMTs) and demethylases (LSD1 [Lysine-specific histone demethylase 1] and JmjC [Jumonji-C] families), along with histone acetyltransferases (HATs) and deacetylases (HDACs), are recognized as key modulators of cell reprogramming. These enzymes alter the genome-wide distribution of repressive and permissive histone marks, promoting either open or closed chromatin states[104]. Methylation of histones occurs at different lysine residues in histones H3 and H4; specifically, H3 methylation at K9 and K27 is generally associated with gene silencing, whereas active genes are linked to methylation at K4 and K36[105].
To investigate the role of histone-tail PTMs in microspore embryogenesis, induction of T. aestivum microspore embryogenesis with mannitol and TSA resulted in hyperacetylation of H3.2, with TSA demonstrating a more pronounced effect. These treatments differentially impacted histone PTMs, underscoring the complex role of acetylation[106]. In addition, inhibitors of histone methylation (chaetocin) and histone phosphorylation (aurora kinase inhibitor II [AUK-II]) were tested across various cultivars, with both chaetocin and AUK-II significantly increasing the percentage of embryogenic structures; notably, AUK-II outperformed TSA[107]. In B. oleracea, the histone deacetylase inhibitor suberoylanilide hydroxamic acid (SAHA) successfully induced embryogenesis[108]. Furthermore, dimethylation of H3K9 (H3K9me2) increased during microspore reprogramming and embryonic development, peaking in globular and torpedo-shaped embryos. The histone methylation inhibitor BIX-01294 was also found to inhibit microspore embryogenesis in B. napus and H. vulgare, linking this epigenetic mark to cellular differentiation[9]. In contrast to H3K9me2, high levels of acetylated histones were observed in vacuolated microspores before embryogenesis induction. The expression pattern of BnHAT closely aligned with the temporal profiles of acetylated histones during this process[61]. Collectively, these studies demonstrate the crucial involvement of histone acetylation and acetyltransferases in activating cell division and proliferation.
Collectively, these studies signify substantial progress in understanding the epigenetic dynamics that regulate the efficiency of microspore embryogenesis induction, particularly in recalcitrant crops. Although the precise relationship between epigenetic modifications, transcriptional changes, and microspore embryogenesis induction remains largely unknown, analyzing epigenetic dynamics in coordination with gene regulation offers new insights into the mechanisms of microspore reprogramming toward embryogenesis, which is still in its infancy, will certainly advance in the near future.
-
Since the first successful production of haploid embryos from Datura anther cultures in 1964[53], in vitro systems for microspore embryogenesis have been developed for hundreds of plant species across various families, although efficiency varies significantly among them[5]. Microspore embryogenesis has been successfully applied in major crops such as wheat (T. aestivum)[109], barley (H. vulgare)[110], maize (Zea mays)[111], and rape (B. napus)[112]. Despite its widespread application for DH production, microspore embryogenesis remains highly inefficient or even completely ineffective for many economically important crop species.
The efficiency of microspore embryogenesis in T. aestivum is significantly genotype-dependent. Key limitations include the low number of microspores that successfully undergo developmental reprogramming, the scarcity of high-quality embryos, and the elevated percentage of albino plantlets. However, many genotypes remain unresponsive to microspore embryogenesis. A major challenge is the low rate of spontaneous chromosome doubling, necessitating the use of toxic agents such as colchicine and orizalin, which result in the loss of DH lines and require an extra seed multiplication cycle[107]. Currently, anther culture is a more reliable method for producing doubled haploids in T. aestivum compared to isolated microspore cultures, which tend to yield more albino plantlets[113].
H. vulgare serves as ideal cereal model for molecular studies on microspore embryogenesis due to its diploid genome, low basic chromosome number of seven, and considerable diversity in microspore-derived plant regeneration capabilities[114]. The size of the resulting embryogenic microspore fraction is significantly influenced by three primary factors: genotype, harvest stage, and type of pretreatment (e.g., its intensity and duration). For specific genotypes and culture conditions, optimizing pretreatment variables to enhance the yield of embryogenic microspores per spike is always desirable[115].
In Z. mays microspore embryogenesis, significant advancements have been made over the past 20 years; yet several challenges remain. Genotype plays a crucial role in androgenesis responsiveness, with elite germplasm often exhibiting low or no response. Key steps in the process, such as plant regeneration from androgenic embryos and spontaneous chromosome doubling, remain inadequately addressed. While recent studies have advanced our understanding of early cellular and molecular events, effectively applying this knowledge to recalcitrant genotypes remain inadequately addressed. Despite the successful culture protocols, microspore culture has not yet become a major tool in maize breeding. Nevertheless, Vergne & Gaillard recently provide a protocol for isolating maize microspores for DH production[116].
In B. napus microspore embryogenesis, significant challenges remain despite advancements. Variability in embryo yield among genotypes emphasizes the need to understand the underlying mechanisms influencing microspore competence. Different embryogenic structures exhibit varying viability and developmental fates, with some transitioning into less embryogenic forms. Cell wall composition significantly impacts these structures, as variations in components such as pectin and callose correlate with embryogenic potential. Moreover, HDAC inhibition can promote certain aspects of embryogenesis but may have detrimental effects, particularly in high-responding genotypes such as DH4079[34]. This highlights the complexity of stress signaling pathways and their differential responses among genotypes, necessitating tailored approaches to enhance microspore embryogenesis across diverse Brassica species.
In summary, the donor plant genotype is particularly crucial for microspore embryogenesis in crop breeding. Other limitations, such as culture conditions and the composition of the culture medium, also affect efficiency[117,118]. For instance, the NLN medium supplemented with 130 g·L−1 sucrose was effective in inducing microspore embryo production in Chinese flowering cabbage (Brassica rapa)[119,120]. These findings underscore the importance of optimizing culture conditions and understanding the underlying mechanisms of microspore embryogenesis to overcome existing limitations. Addressing these challenges can significantly expand the potential application of microspore embryogenesis, enhancing its utility in crop improvement and breeding programs.
-
Microspore embryogenesis is a valuable technique in plant research and plant breeding, providing a powerful means to generate DH plants and offering unique insights into cellular reprogramming as well as the acquisition of totipotency (Fig. 1). This technique leverages stress treatments, such as heat shock, to redirect the developmental pathway of microspores—normally destined to become pollen grains—towards embryogenesis. However, the application of microspore embryogenesis is not without its challenges. The process often suffers from inefficiencies, including low induction rates, high levels of cell death, and the difficulty in distinguishing between responsive and non-responsive microspores. Overcoming these limitations will require a deeper understanding of the underlying mechanisms governing microspore reprogramming, stress response, and cell fate determination. Future research aimed at unraveling the molecular and biochemical pathways that regulate microspore embryogenesis will be crucial for addressing these challenges. Improvements in our ability to manipulate cytoskeleton dynamics, cell wall remodeling, programmed cell death, autophagy, and hormonal responses may lead to more efficient protocols, paving the way for broader application across a range of plant species. These advancements hold great promise for accelerating breeding programs and enhancing crop improvement efforts worldwide.
Figure 1.
A schematic of the process of microspore embryogenesis. In the natural gametophytic developmental pathway, the vacuolated microspore undergoes asymmetrical division to produce a bicellular pollen grain, which matures further into a tricellular pollen grain. However, when isolated microspores are subjected to stress in vitro, they undergo reprogramming. This cell reprogramming leads to the microspores dividing to form proembryos, which then develop into full embryos. Several determinant factors govern this process, including cytoskeleton, cell wall remodeling, programmed cell death, autophagy, hormonal responses, and epigenetic modifications. The remaining microspores typically undergo cell death. V, vegetative nucleus. G, generative nucleus. S, sperm nucleus.
-
While microspore embryogenesis presents substantial potential for advancing plant breeding and research, several challenges must be addressed to enhance its efficiency and unravel its underlying mechanisms. Despite recent progress in molecular biology, much of the research remains focused on cellular descriptions using in vitro treatments over extended periods. Therefore, deeper investigations using advanced molecular and cellular techniques are crucial to addressing the following key questions:
(i) Novel mechanisms of stress-induced microspore cell fate transition: Beyond the mechanisms discussed so far, what additional pathways or molecular signals contribute to the reprogramming of microspores under stress conditions? Identifying novel regulatory networks could provide new insights into how microspores transition to an embryogenic development.
(ii) Reliable molecular markers for early selection of embryogenic microspores: What molecular markers can be identified that reliably distinguish embryogenic microspores at the earliest stages? Early detection of embryogenic microspores could significantly improve the induction efficiency by allowing for the selective cultivation of these cells.
(iii) Genes activated or inhibited by epigenetic modification: Which specific genes are de novo activated or repressed by epigenetic modifications during the initiation of microspore embryogenesis? Understanding how epigenetic changes influence gene expression at the onset of embryogenesis could reveal targets for enhancing the process.
(iv) Innovative methods to increase microspore embryogenesis frequency: What novel approaches could be developed to significantly and broadly enhance the frequency of microspore embryogenesis, particularly for species that are currently recalcitrant to this technique? Innovative strategies might include the use of new chemical treatments, gene editing techniques, or optimized culture conditions.
(v) The comparative study between zygotic and microspore embryogenesis: Comparative analyses can reveal significant similarities between zygotic and microspore embryogenesis. Identifying and validating of determinant factors that influence embryogenesis may have implications for other forms of embryogenesis. The application of these regulators could be instrumental in reducing cell mortality and enhancing the rate of embryogenesis, particularly in in vitro settings. Furthermore, using microspore embryogenesis as a model to study the initiation of embryogenesis could provide insights into the complexities of zygotic embryogenesis, which is deeply buried in maternal tissues and difficult to access[75].
Exploring the unanswered questions and overcoming the challenges associated with microspore embryogenesis will deepen our understanding of this complex process and enhance its practical applications in plant breeding. Addressing the obstacles related to low efficiency, high cell death rates, and species-specific limitations is essential for unlocking the full potential of microspore embryogenesis as a reliable tool for producing DH plants. These advancements could accelerate the development of new plant varieties with desirable traits, significantly contributing to agricultural innovation and productivity. By refining techniques and optimizing regulatory mechanisms, microspore embryogenesis could become more effective, enabling its broader application across a wider range of crops species, ultimately supporting global food security and sustainable agricultural practices.
This work was supported by the National Natural Science Foundation of China (32000248).
-
The authors confirm contribution to the paper as follows: draft manuscript preparation: Yang F, Liu X, Qiao Y, Tang X, Luo P. All authors approved the final version of the manuscript.
-
Data sharing not applicable to this review as no datasets were generated or analyzed during the current study.
-
The authors declare that they have no conflict of interest.
-
# Authors contributed equally: Fan Yang, Xinyu Liu, Ying Qiao
- Copyright: © 2024 by the author(s). Published by Maximum Academic Press on behalf of Hainan Yazhou Bay Seed Laboratory. This article is an open access article distributed under Creative Commons Attribution License (CC BY 4.0), visit https://creativecommons.org/licenses/by/4.0/.
-
About this article
Cite this article
Yang F, Liu X, Qiao Y, Tang X, Luo P. 2024. Microspore embryogenesis: in vitro cultivation induced cell reprogramming for plant breeding. Seed Biology 3: e021 doi: 10.48130/seedbio-0024-0019
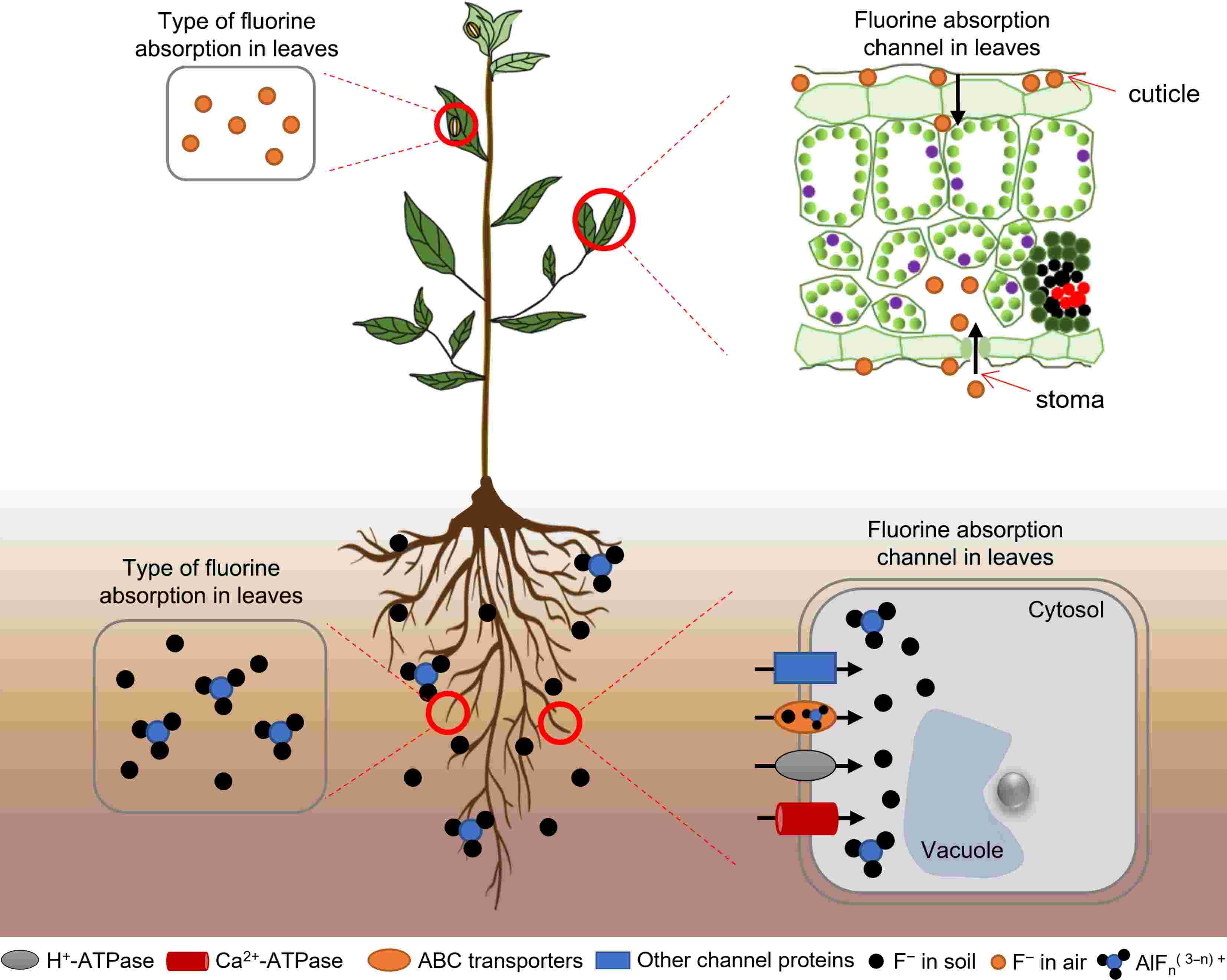