-
The toxicity of polycyclic aromatic hydrocarbons (PAHs), such as their mutagenic and carcinogenic effects, has raised concerns regarding their behavior and movement in the environment (Kastner et al., 1998; Hamdi et al., 2007). These persistent organic pollutants are closely associated with vegetation, and there is potential for accumulation in living organisms. Consequently, vegetation plays a vital role in these chemicals' worldwide distribution and environmental destiny (Barber et al., 2004). To diminish their impact, it is crucial to comprehend the mechanisms by which PAHs infiltrate, persist, and become active within plants.
For over a decade, researchers have conducted numerous studies demonstrating the absorption of PAHs by the roots of diverse plant species from contaminated soils, followed by their transportation to the shoots (Abhilash et al., 2009; Alagić et al., 2015; Wu et al., 2019; Shen et al., 2020). Initially binding to the epidermis in the elongation zone, PAHs taken up by plant roots subsequently traverse the epidermal cells to reach the cortex through both apoplastic and symplastic pathways (Wild et al., 2005). The subcellular distribution of lipophilic PAHs in ryegrass roots can be influenced by the lipid content of intracellular components and transpiration (Kang et al., 2010). Research investigating the uptake of phenanthrene (Phe) by Medicago sativa in the presence of an arbuscular mycorrhizal fungus found that inoculating lucerne with the fungus Glomus etunicatum L. led to increased Phe accumulation (Wu et al., 2009). Furthermore, Shen (2020) provided evidence for the temperature-dependent symplastic and apoplastic pathways facilitating the phloem-mediated acropetal translocation, transfer, and accumulation of PAHs in wheat leaves.
Plant growth and development are primarily limited by phosphorus (Marschner, 1995). The active absorption of phosphorus occurs through phosphate (Pi) transporters in the rhizosphere (Raghothama, 1999). It has been demonstrated that the increase of P concentration in wheat roots inhibited Phe uptake (Yang et al., 2016). LPR1 and LPR2 (LOW PHOSPHATE ROOT) in Arabidopsis were identified to be the central roles in local Pi sensing in the plant roots (Reymond et al., 2006; Ticconi et al., 2004, 2009; Svistoonoff et al., 2007). We had identified the expression patterns of all five rice OsLPR family members, the homologs of LPR1/2, and in the roots, quantitative real-time RT-PCR (qRT-PCR) analysis showed elevated expression levels of OsLPR3 and OsLPR5, with a significant increase observed under Pi deficiency (Cao et al., 2016). Using the generated OsLPR5-overexpressors and CRISPR-Cas9-mediated OsLPR5-mutants (oslpr5-1 and oslpr5-2), we subsequently provided empirical evidence for the broad-spectrum role of OsLPR5 in developmental responses and the maintenance of Pi homeostasis (Ai et al., 2020).
In the present study, we investigated the role of OsLPR5 in regulating Phe (a model PAH) accumulation and distribution in rice, focusing on the influence of Pi supply. Phe uptake was significantly reduced in oslpr5-1 and oslpr5-2 mutants compared to the wild type under Pi-deficiency conditions, as observed in hydroponic and soil experiments. Moreover, the mutation of OsLPR5 led to increased Phe allocation in rice seeds. These findings offer a new understanding of Phe accumulation in higher plants and propose an effective agronomic approach to reduce crop contamination.
-
Phenanthrene (Phe) of over 95% purity was procured from Aladdin Industrial Company (Shanghai, China). HPLC-grade methanol was used throughout the research. Wild-type rice (Oryza sativa L. ssp. japonica cv. Nipponbare) and OsLPR5-mutant lines (oslpr5-1 and oslpr5-2) derived from our prior investigation (Ai et al., 2020) were utilized.
The seeds of wild-type and mutants of OsLPR5 (oslpr5-1 and oslpr5-2) were surface-sterilized with the solution which was prepared with NaClO solution (30% NaClO, commerial product) and water (1:3, v/v) for 30 min. Afterward, they were washed with deionized water. The sterilized seeds were germinated in the dark at 37 °C for 4 d and then transferred to a hydroponic foam floating plate. Finally, the plants were placed in a growth chamber, inside a glass jar filled with aerated half-strength Hoagland's solution.
To conduct the hydroponic experiments, consistent growth seedlings of wild-type (WT) and oslpr5 lines were selected (14 days old). These seedlings were transferred to glass tanks containing 8 L of Hoagland's solution, adjusted to a pH value of 5.5 using a buffer solution called MES (2-[N-Morpholino] ethane sulfonic acid). The Hoagland's solution should be supplemented with varying concentrations of Phe (stock solution prepared with methanol as the solvent) and phosphate. Eight days after seedling treatment, plant samples were collected to measure the concentration of phenanthrene and RNA was extracted. For the two experiments, the concentrations of Phe are 0, 0.5, 1, and 1.5 mg/L.
For another set of hydroponic experiments, two different concentrations of Pi were used in Hoagland's solution: 200 μM Pi (+P) and 10 μM Pi (−P). In the -P group, KH2PO4 was used for phosphorus treatment, and 200 μM KCl was added to maintain the potassium concentration consistent with that of the normal rice nutrient solution, while the other nutrients remained the same as in the normal rice nutrition liquid. Additionally, two variations of Hoagland's solution were prepared: one treated with 1.5 mg/L Phe (dissolved in methanol), and the other without Phe. The seedlings were subjected to a 35-d treatment period, during which Hoagland's solution was refreshed every 2 d. The hydroponic system's media was changed every 2 d, and the seedlings were kept in a growth room under conditions identical to those described in Ai et al. (2020).
For the pot experiments, soil samples were obtained from the Nanjing Agricultural University Experimental Farm (Nanjing, China), and collected at a depth of 0–20 cm above the field surface. The available P concentration in the soil is 26.7 mg/kg. To ensure the even distribution of Phe in the soil, the collected soil was dried, cleared of plant debris, and crushed. It was then dissolved in acetone and added to the soil, followed by mixing with a blender. Continuous stirring of the soil facilitated acetone evaporation. The initial Phe concentration in the soil was 60 mg/kg (+Phe). In order to enhance soil nutrient levels, urea (300 mg/kg soil), KH2PO4 (20 mg/kg soil), and K2SO4 (200 mg/kg soil) were applied. Subsequently, 15 kg of soil was transferred to each pot, and each pot was planted with one wild-type plant and two mutant plants of 21-d seedling age. Three replicates were conducted for each experiment.
Rice and soil sampling for Phe analysis
-
Wild-type and OsLPR5-mutant lines were cultivated using Hoagland's solution for 8 d (14-day-old seedlings) and soil pots for 20 weeks (21-day-old seedlings) with varying Phe concentrations. The processing method of plant samples at harvest time is described by Jiao et al. (2007). The collected plant samples were subsequently divided into two categories: hydroponic samples, consisting of roots and shoots, and soil-grown samples, comprising culms, flag leaves, and seeds. Surface samples from the top 20 cm layer of the soil were collected for potting soil experiments. The plant and soil samples were freeze-dried and stored at −20°C in a refrigerator.
Analysis of Phe in soil and plant tissues
-
To measure Phe concentrations in soil and plants, dry samples weighing approximately 2 and 0.5 g were utilized, following a previously described method (Wang et al., 2021).
Quantification of total Pi concentration and qRT-PCR
-
The three-week-old wild-type and OsLPR5-mutant lines (oslpr5-1 and oslpr5-2) were cultivated in potted-soil for a period of 20 weeks, followed by a 35-d hydroponic experiment. The harvested tissues were then quantified for total Pi concentration using the Kjeldahl method, as previously described by Bradstreet (1954).
The RNA extraction methods were performed according to Ai et al. (2020). The 2−ΔΔCᴛ method of relative quantification (Livak & Schmittgen, 2001) was used to calculate the relative expression levels of the genes. Specific primers for the genes can be found in Supplemental Table S1.
Statistical analysis
-
The data obtained from four independent biological experiments were computed and analyzed using the IBM SPSS Statistics 20.0 software. One-way ANOVA (Duncan's test) with multiple comparisons was employed to detect significant differences.
-
The acquisition of Phe by the roots and its subsequent translocation to the shoots have been demonstrated in ryegrass and wheat (Gao et al., 2004; Shen et al., 2020). To test the acquisition and translocation of Phe in rice, the seedlings of wild type (WT) were hydroponically grown in the solution supplemented with the different Phe concentrations. As shown in Fig. 1a, there were slightly dosage-dependent inhibitory effects on the plant length, but no big difference in the biomass of shoot and root (Fig. 1b). The Phe concentrations in both roots and shoots at the gradient Phe treatment level were assayed. There were significant dosage-dependent increases in the Phe concentration in the roots and shoots concomitant with the increase in Phe concentration in the medium after the treatments (Fig. 1c). These findings align with our previous study, which demonstrated significant increases in Phe concentration in rice roots and shoots, correlating with the duration and Phe concentration in the medium (Wang et al., 2021). Across all Phe treatment concentrations, the concentration of Phe in the roots was significantly higher than in the shoots, ranging from 39.72−280.78 μg/g in roots and 4.49−12.77 μg/g in shoots. This suggests that Phe is transported acropetally in rice seedlings (Fig. 1c).
Figure 1.
Effects on the growth and Phe accumulation under different Phenanthrene concentration treatments in rice. WT seedlings, aged 14 d, were hydroponically grown in Hoagland's solution (pH 5.5) with varying concentrations of Phenanthrene (Phe) supplementation for a duration of 8 d. (a) The growth of WT seedlings was evaluated to observe the phenotypes resulting from different concentrations of Phe. The scale bar is 20 cm. (b) Biomass of roots and shoots as described in (a). Data are presented for the concentrations of phenanthrene in roots and shoots (c). Means ± SE values are reported (n = 6), and significant differences are denoted by different letters on the histograms (p < 0.05, one-way ANOVA).
Phosphate is involved in the uptake and translocation of phenanthrene in rice
-
Under various treatments of Pi, the WT seedlings were hydroponically grown in Hoagland's solution supplemented with Phe. The analysis indicated that the Phe concentration was much higher in the roots than those in the shoots at +P and −P conditions by 0.86 and 10.97 times, respectively. The concentrations of Phe were induced significantly by the Pi-deficient supply in both the roots and shoots. In comparison with +P, −P contained 14.62 and 1.43 times higher Phe concentrations in the roots and shoots, respectively (Fig. 2). This is consistent with the results that wheat phosphate-deficiency can promote the asportation and accumulation of Phe, and the increase of P concentration inhibited Phe uptake (Yang et al., 2016).
Figure 2.
The impact of different phosphorus treatments on Phe accumulation in WT. Under Pi-sufficient (+P [200 μM Pi]) and Pi-deficient (−P [10 μM Pi]) conditions, the wild-type (WT) seedlings were grown hydroponically with 1.5 mg/L phenanthrene for 35 d. Data are presented for the concentrations of phenanthrene in roots and shoots of WT. Means ± SE values are reported (n = 4), and significant differences are denoted by different letters on the histograms (p < 0.05, one-way ANOVA).
Phosphate deficiency-induced OsLPR5 and OsLPR3 were responsible for phenanthrene in rice
-
Low Phosphate Root1 (LPR1; At1g23010) in Arabidopsis encodes multi-copper oxidase, and there are five homologs in rice. In our previous study, five Low Phosphate Root in rice were identified, of which OsLPR5 and OsLPR3 (OsLPR5/3) displayed the significant induction at Pi deficient condition (Cao et al., 2016). We speculated that OsLPR5/3 might be involved in the phosphate-mediated accumulation of Phe in rice. To validate this, qRT-PCR was employed to detect the dosage-dependent spatiotemporal effects of Phe on the expression pattern of OsLPR5/3 in the seedling. As shown in Fig. 3, the expression levels of OsLPR5/3 were much greater in the roots than those in the shoots of the seedlings under Phe. High expression levels of OsLPR5/3 in the roots suggested their potentially important roles in the acquisition of Phe by the roots from the rhizosphere, which is similar to the trend of LPR family numbers on the Pi uptake in Arabidopsis roots (Svistoonoff et al., 2007; Ticconi et al., 2004, 2009).
Figure 3.
Relative expression levels of (a) OsLPR3 and (b) OsLPR5 in response to phenanthrene in the roots and shoots of rice. The WT seedlings were subjected to phenanthrene treatments at different concentrations (0, 0.5, 1, and 1.5 mg/L) for a duration of 9 d. Means ± SE values are reported (n = 4), and significant differences are denoted by different letters on the histograms (p < 0.05, one-way ANOVA).
Mutation of OsLPR5 changes the root development under different Phe and Pi levels
-
In our previous study, the knockout of OsLPR5 by means of CRISPR-Cas9 resulted in adverse effects on Pi acquisition (Ai et al., 2020). Therefore, we investigated firstly the effects of OsLPR5 on the accumulation of Phe, and the roles of Pi in this process in this study. Two independent transgenic lines with the mutation of OsLPR5 (Ai et al., 2020) were used in the experiments after the molecular identification for them (Supplemental Fig. S1), and hereafter referred to as oslpr5-1 and oslpr5-2.
The effect of PAHs on plant growth has been well investigated (Kummerova et al., 2004; Váňová et al., 2009; Kummerova et al., 2013). To understand whether the mutation of OsLPR5 affects the phenotypic traits of rice seedlings with Phe under different Pi supplies, WT and oslpr5 seedlings were grown hydroponically in Hoagland's solution supplied with either +Phe and +P, +Phe and −P, −Phe and +P, or without both Phe and P. The root length phenotypic traits were documented after the treatments (Fig. 4). Compared with the corresponding WT plants, the ratios of relative root length (−P/+P; +Phe/−Phe) in oslpr5 lines were both comparable under −Phe and +P, respectively. However, the corresponding ratios exhibited a significant decrease by 16.21%−20.12% and 17.03%−20.31% under +Phe and −P conditions, respectively, compared with the WT plants (Fig. 4b, c). These results indicated that Phe exposure impaired the growth of the root length in the oslpr5 mutants, while phosphate was involved in this process under Pi- deficiency.
Figure 4.
Effects of mutation in OsLPR5 on the phenotype, and length of root during growth under different regimes of Phe and Pi. The 14-day-old seedlings, including the WT and OsLPR5 knockout mutants (oslpr5-1 and oslpr5-2), were grown hydroponically for a period of 35 d. The growth conditions included supplementation with −Phe (0 mg/L) and +Phe (1.5 mg/L), and the plants were subjected to both +P (200 μM Pi) and −P (10 μM Pi) conditions. (a) The phenotype of the seedlings. Scale bar = 10 cm. Data are presented for (b) Ratio (−P/+P) and (c) Ratio (+Phe/−Phe) of root length, respectively. Means ± SE values are reported (n = 4), and significant differences are denoted by different letters on the histograms (p < 0.05, one-way ANOVA).
Mutation of OsLPR5 decreased Phe uptake and increased Phe translocation significantly under Pi-deficiency in hydroponic experiments
-
Concomitant with the increase in Phe concentration in the medium, the expression levels of OsLPR5 in the root were significantly induced (Fig. 3). It was logical to investigate if OsLPR5 regulates Phe acquisition and accumulation in rice, and phosphate is involved in this process. For this, the rice seeds were grown after germination in a nutrient solution supplied with P and Phe (+P+Phe), low P and Phe (-P+Phe). As shown in Fig. 5, Phe concentrations of the roots in oslpr5-1 and oslpr5-2 were comparable with those of WT at +P+Phe, indicating that the mutation of OsLPR5 did not affect the uptake of Phe in this case. However, it clearly showed that Phe concentrations in oslpr5-1 and oslpr5-2 were significantly lower compared with WT, by 36.45% and 30.96%, respectively, at −P+Phe (Fig. 5a). Correspondingly, in the shoots, there are no big differences at Phe concentrations between oslpr5 and WT at both +P+Phe and −P+Phe (Fig. 5b). Then the translocation factor (TF) of Phe were calculated. TFs of oslpr5 were comparable with those of WT under +P+Phe, but there was a significant increasing trend in TF, and it was higher by 74.99%−93.36% in oslpr5 than those of WT (p < 0.05) under −P+Phe (Fig. 5c). These results indicated that the mutation of OsLPR5 decreased Phe absorption and increased Phe translocation under Pi-deficiency.
Figure 5.
The mutation in OsLPR5 has an impact on the acquisition and translocation of Phenanthrene (Phe) under different phosphorus (Pi) regimes. The 14-day-old WT and oslpr5 seedlings were grown following the instructions provided in the legend of Fig. 4. Data are presented for the concentrations of phenanthrene in (a) roots and (b) shoots and (c) translocation factor. Means ± SE values are reported (n = 4), and significant differences are denoted by different letters on the histograms (p < 0.05, one-way ANOVA).
Mutation of OsLPR5 reduced the absorption of Phe in oslpr5 under soil experiments
-
It had been exhibited that the mutation of OsLPR5 resulted in the change of Phe absorption and accumulation in oslpr5 compared with WT under −P condition in Fig. 5. To understand the effects of the mutation of OsLPR5 on Phe hemostasis under Phe in the whole growth period of rice, the WT and oslpr5 (21-day-old) were grown to maturity in a potting soil supplemented with 60 mg/kg Phe for 20 weeks. As shown in Fig. 6, compared with WT, the concentrations of Phe were significantly decreased in oslpr5, accumulating 3.24 and 3.32 mg/kg Phe in the culms and flag leaves, being 40.71% and 20.57 % lower than WT on average, respectively, under Phe (Fig. 6a, b). However, Phe concentration was significantly higher, being 72.89% in the seeds of oslpr5 lines than that in the WT (Fig. 6c). The results revealed the mutation of OsLPR5 resulted in the decrease of the vegetable organs and the increase of the reproductive organ of oslpr5.
Figure 6.
Concentrations of phenanthrene of different tissues in wild-type and oslpr5 in pot experiments grown to maturity. The 21-day-old seedlings, both the WT and oslpr5, were grown in potting soil supplemented with 60 mg Phe/kg soil for a period of 20 weeks. Phenanthrene concentrations of wild-type and oslpr5 were measured and data are presented for (a) culms, (b) flag leaf, and (c) seeds. Means ± SE values are reported (n = 4), and significant differences are denoted by different letters on the histograms (p < 0.05, one-way ANOVA).
-
Phosphorous is an essential nutrient necessary for plant growth and development. Moreover, it has been demonstrated that there is a relationship between the concentrations of Pi and Phe in wheat (Yang et al., 2016). Therefore, it reasoned to characterize the effect of phosphate (Pi) on the acquisition and translocation of Phe in rice. The results of the present study showed that phosphate is involved in the uptake and translocation of Phe in rice (Fig. 2). Nitrogen is a key component of the nucleotides and proteins, and a key element for plant growth (Xu et al., 2012). It has also been documented that nitrogen affected Phe uptake by the roots and Phe translocation from the root to shoot in lettuce and wheat seedlings (Zhan et al., 2015). These results indicate that the essential nutrients (Phosphorous and Nitrogen) affect the asportation and transport of Phe in plants.
OsLPR5 was abundantly expressed and responsible to Pi in the roots, but there were few and no significant differences in the shoots of rice (Cao et al., 2016). Concomitant with the increase in Phe concentration in the medium (0.5, 1 mg/L), a significant increase in the expression levels of OsLPR5 was observed in the roots. However, the transcript levels of OsLPR5 were comparable in the shoots under the different Phe treatments (Fig. 3). The mutation of OsLPR5 changes the root development under different Phe and Pi levels (Fig. 4). The results suggested that the mediation of OsLPR5 on the accumulation of Phe in rice was accompanied by Pi. Compared with Pi-sufficiency there were significant increases in the expression levels of the gene in the roots of Pi-deficient seedlings in our previous work (Cao et al., 2016). The mutation of OsLPR5 increased Phe translocation significantly under Pi-deficiency (Fig. 5), implying that OsLPR5 is involved in the acquisition of Phe by regulating the uptake of Pi in rice.
Under Pi deficiency, a higher expression level of OsLPR5 was detected in the roots (Cao et al., 2016). Knockout mutation of OsLPR5 using CRISPR-Cas9 resulted in adverse effects on Pi translocation (Ai et al., 2020). In the present study, OsLPR5 is involved in the regulation of the homeostasis for Phe in rice. The mutation of OsLPR5 decreased Phe uptake and increased Phe translocation significantly in oslpr5 in hydroponic experiments (Fig. 5). The concentration was significantly higher in the seeds of oslpr5 than that in the WT under soil experiments (Fig. 6). It has been indicated that OsLPR5 played the role to inhibit the allocation of Phe from the vegetable organ to the seeds in rice. Also, it has been demonstrated that in the seeds of the overexpressing OsNRT2.3b lines, Phe concentration was significantly lower compared with the wild type (Wang et al., 2021). Therefore, the study revealed the function of OsLPR5 in mitigating the Phe toxicity by reducing its entry into the seeds.
-
The wild-type seedlings exhibited a big induction in Phe concentrations under -P, especially in the roots. Expression of Pi-responded OsLPR5 by quantitative real-time RT-PCR was induced significantly under Phe in the roots. The phenotypic traits exhibited the differential changes in oslpr5 compared with the corresponding seedlings grown with Phe under the different phosphate levels. Compared with the WT, the ratios of relative root length (−P/+P; +Phe/−Phe) in oslpr5 lines were both comparable under −Phe and +P. However, the corresponding ratios exhibited a significant decrease under +Phe and −P conditions. Compared with the WT, Phe concentration significantly decreased in the roots of oslpr5 lines under Pi-deficiency. It is possible that mutation of OsLPR5 is useful for breeding low Phe accumulating cultivars of rice grown in Pi-sufficient soil.
-
The authors confirm contribution to the paper as follows: study conception and design: Sun S; data collection: Cui M, Zhao C, Lin Q, Sun Y, Shen X, He P, Yang S, Huang X, Hu Z; analysis and interpretation of results: Cui M, Zhao C, Wang X, Cao Y; draft manuscript preparation: Sun S, Cui M, Wang X.
-
Sequence data from this article can be found in the Rice Genome Initiative or GenBank/EMBL libraries under the following accession numbers: OsLPR3 (LOC_Os01g03630), OsLPR5 (LOC_Os01g03640) and OsActin1 (LOC_Os03g50885).
This work was partially supported by the National Key Research and Development Program of China (2021YFF1000404), National Natural Science Foundation of China (42107428), Scientific and Technological Planning Project of Guangdong, China (2021B1212040008, 20210502). We thank Prof. Ajay Jain, Amity University Rajasthan, India, for his great help in writing the manuscript.
-
The authors declare that they have no conflict of interest.
- Supplemental Table S1 Gene-specific primers used for quantitative RT-PCR.
- Supplemental Fig. S1 Identification of oslpr5-1 and oslpr5-2.
- Copyright: © 2023 by the author(s). Published by Maximum Academic Press, Fayetteville, GA. This article is an open access article distributed under Creative Commons Attribution License (CC BY 4.0), visit https://creativecommons.org/licenses/by/4.0/.
-
About this article
Cite this article
Cui M, Zhao C, Lin Q, Wang X, Sun Y, et al. 2023. Phosphate deficient-induced OsLPR5 mediates the accumulation and distribution of phenanthrene in rice under Pi-deficiency. Soil Science and Environment 2:8 doi: 10.48130/SSE-2023-0008
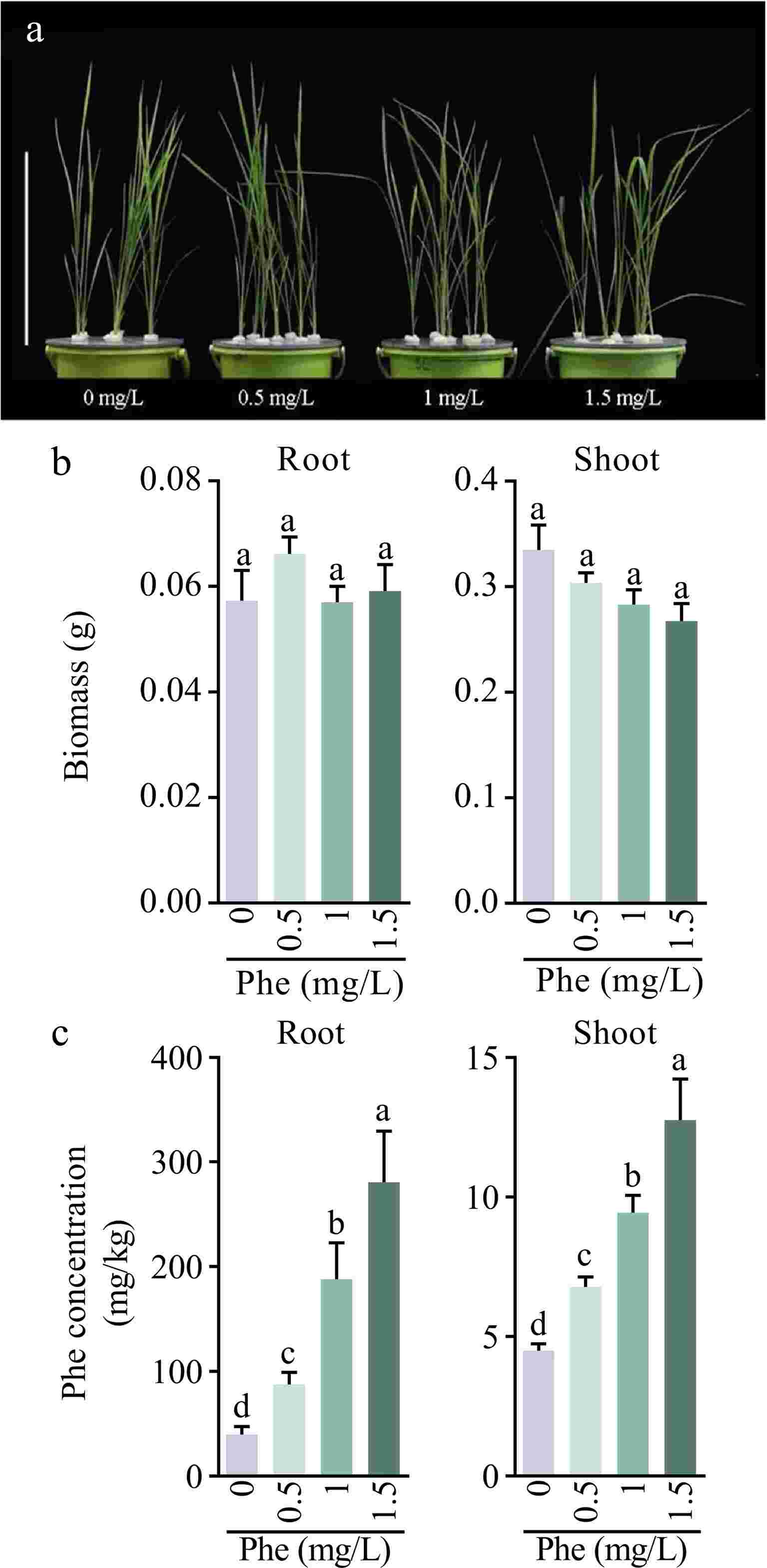