-
The majority of terrestrial plants have plant epidermal trichomes, a specific structure of epidermal cells that extends from the outside of organs, including leaves, stems, flowers, and fruits[1]. Plant trichomes act as a natural barrier between plants and their surroundings, keeping pathogens, insects, herbivores, and other invaders out. Plants utilize it as their first line of defense against outside invasion[2,3]. Trichomes vary in size, shape, number of cells, origin, location, function, etc. They can be divided into long and short types based on their morphological characteristics[4]. Based on the shape of the glandular head cells, they can be categorized as stellate, chained, squamous, etc. Additionally, trichomes are classified into non-secretory glandular trichomes and secretory glandular trichomes based on their secretion function[5]. Many plants, including A.annua, Cannabis sativa L., Mentha haplocalyx Briq., Pogostemon cablin (Blanco) Benth., Perilla frutescens (L.) Britt., and Nicotiana tabacum L., have secretory or non-secretory glandular trichomes[6−11].
A unique, glandular-like appendage that develops in the epidermal tissue of aboveground organs in plants is referred to as a non-secretory glandular trichome. Non-secretory glandular trichomes have various functions, including stress tolerance and defense[1,12], and they shield plant growth and organs. Plants use it as a strong defense against both biotic and abiotic stress. Malvaceae plants, such as cotton[13], and Brassicaceae plants, including Arabidopsis[14], both have a large distribution of non-secretory glandular trichomes. Secretory glandular trichomes, being observed on approximately 30% of all vascular plant species[15], are characterized by their metabolic capacity to produce, store, and secrete copious secondary metabolites and thus earn them the moniker 'biosynthetic factories'[16]. Some plant secretory glandular trichomes, from the Asteraceae, Lamiaceae, Cannabaceae, and Solanaceae families have been found to contain natural compounds such as terpenoids, alkaloids, phenols, and flavonoids[17,18].
A. annua, a member of the Asteraceae family and an annual herb of the Artemisia genus, possesses a bitter and pungent taste and is characterized by its cold and cool properties. It effectively clears deficiency heat, eliminates bone steaming, relieves heat, and intercepts malaria[19]. The glandular trichomes of A. annua predominantly occur on the leaves, flowers, and flower buds[20]. Notably, the secretory glandular trichomes of A. annua serve as the primary site for the production and accumulation of artemisinin[20−22]. Artemisinin is one of the most effective drugs for the treatment of malaria, and artemisinin-based combination therapies (ATC) are the most effective and important means of treating malaria nowadays[23,24]. In addition to their classical antimalarial effects, artemisinin and its derivatives exhibit a wide range of biological activities, including antibacterial, antioxidant, anti-inflammatory, antiviral, anticancer, and cardioprotective effects[25−28]. Recent scientific studies have revealed that artemisinin derivatives are highly effective in improving the disease manifestations of polycystic ovarian syndrome (PCOS), a discovery that opens new avenues for the treatment of PCOS[29]. These diverse pharmacological effects indicate that artemisinin and its derivatives have great potential and broad application prospects for assisting in the treatment of various diseases and the development of new drugs. Artemisinin, a significant contribution of traditional Chinese medicine to the world serves as a frontline antimalarial drug, saving millions of lives annually. With the emergence of new indications, the market demand for artemisinin is increasing. The content of artemisinin is tightly associated with its secretory glandular trichomes. Nevertheless, secretory glandular trichomes are prone to lose and make up only 2% of the dry weight of the plant. Only 0.01% to 0.8% of the dry weight of A. annua plants consists of artemisinin[30]. In the face of the broad prospects for the clinical application of artemisinin and its growing market demand, how to effectively increase its production has become an important issue that needs to be tackled by the scientific research community and the industry. This review systematically elaborates on the morphology and function of A. annua glandular trichomes, factors affecting the growth and development of glandular trichomes, and the synthesis mechanism of artemisinin in secretory glandular trichomes to promote the cultivation of high-quality A. annua varieties and improve artemisinin production.
-
The appearance, size, and cell content of the many types of glandular trichomes in A. annua vary, and observations of these trichomes date back to the 20th century. There are two types of glandular trichomes in A. annua, namely glandular secreting trichomes (AaGSTs)and T-shaped non-glandular trichomes (AaTNGs), both of which are multicellular head-shaped glandular trichomes[31,32] (Fig. 1). These two varieties of glandular trichomes are mainly distributed in the leaves, flowers, stems, buds, and other parts of A. annua, and play a specific biological function[33,34].
Figure 1.
Morphological characteristics of trichomes in A. annua. AaGST, glandular secreting trichome; Ba, basal cells; St, stalk cells; Suc, subapical cells; Ac, apical cells; SS, subcutaneous space; AaTNG, T-shaped non-glandular trichome.
AaGST is a 10-cell double-column semi-transparent structure consisting of two basal cells (Ba), two stalk cells (St), four subapical cells (Suc), two apical cells (Ac), and one subcutaneous space (SS)[20,35], can synthesize, secrete, and store rich metabolites such as terpenes, alkaloids, catechol tannins, polysaccharides, and calcium oxalate. Artemisinin is produced in the apical and periapical cells of AaGSTs and accumulates in the subepidermal space of AaGSTs[35,36]. AaGSTs release harmful chemicals to insects and animals, thereby blocking their further damage to plant bodies. Studies have shown that artemisinin has a certain resistance to parasites[37]. Protrusions that separate from plant epidermal cells and do not possess secretory activity are referred to as non-secretory glandular trichomes. AaTNG is a five-cell structure with elongated filamentous cells at the head, also known as T-shaped trichomes because of their similarity to the letter 'T'[20,36,38]. AaTNGs are widely found on the epidermis of leaves, stems, and flowers of A. annua[31], and generally play a protective role for the plant against various biotic and abiotic stresses, with the function of preventing insects from nibbling, preventing low-temperature infestation, and preventing animals from attacking the plant[39].
-
The growth and development of A. annua trichomes are intricately coordinated and regulated by a multitude of factors, including regulatory genes, phytohormones, microRNAs, and the environment (Table 1). Among these factors, regulatory genes including transcription factors (TFs) and functional genes play a dominant role in the initiation, growth, and development of trichomes, whereas both plant hormones and microRNAs finally affect the formation and development of trichomes through the regulation of trichome-related genes (Fig. 2).
Table 1. The main factors regulating the growth and development of A. annua trichomes.
Types Name Effect Target gene Interacting proteins Refs R2R3-MYB AaMYB1 (+)AaGST initiation
(+)artemisinin biosynthesisADS, CYP71AV1 [40] AaMYB5 (−)AaGST formation
(−)artemisinin biosynthesisAaHD1, AaMYB16, AaJAZ8 [41] AaMYB16 (+)AaGST initiation
(+)AaTNG initiation
(+)artemisinin biosynthesisAaHD1, AaMYB5 [41] AaMYB17 (+)AaGST initiation
(+)artemisinin biosynthesis[42] AaMIXTA1 (+)AaGST initiation
(+)AaTNG initiation
(+)artemisinin biosynthesisAaCYP77A1, AaCYP86A1, AaKCS5, AaCER1, AaABCG12 [43] AaTLR1 (−)AaGST formation
(−)artemisinin biosynthesisADS, CYP71AV1, DBR2, ALDH1 AaTLR2, AaWOX1 [44] AaTLR3 (−)AaGST formation
(−)artemisinin biosynthesisADS, CYP71AV1, DBR2, ALDH1 AaCycTL [45] AaTAR2 (+)AaGST initiation
(+)AaTNG initiation
(+)artemisinin biosynthesisADS, CYP71AV1, DBR2, ALDH1 [46] AaMYB108-like (+)AaGST initiation
(+)AaTNG initiation
(+)artemisinin biosynthesisAaHD1 AaHD8 [47] HD-ZIP AaHD1 (+)AaGST initiation
(+)AaTNG initiation
(+)artemisinin biosynthesisAaGSW2 AaJAZ8 [34] AaHD8 (+)AaGST initiation
(+)AaTNG initiation
(+)artemisinin biosynthesisAaCYP86A4, AaFDH AaMIXTA1 [48] AP2/ERF AaTAR1 (+)AaGST formation
(+)AaTNG formation
(+)artemisinin biosynthesisADS, CYP71AV1 AaCycTL [38] AaWIN1 (+)AaGST formation
(+)artemisinin biosynthesisAaGSW2 AaMIXTA1 [49] AaSPL9 (+)AaGST initiation
(+)artemisinin biosynthesisAaGSW2 AaHD1 [50] WRKY AaGSW2 (+)AaGST initiation
(+)artemisinin biosynthesisAaHD1, AaHD8 [51] SAP AaSAP1 (+)AaGST formation
(+)artemisinin biosynthesis[52] LEAFY-like AaTLR2 (−)AaGST initiation
(−)AaTNG initiation
(−)artemisinin biosynthesisADS, CYP71AV1, DBR2, ALDH1 AaWOX1 [44] MADS-box AaSEP1 (+)AaGST initiation
(+)artemisinin biosynthesisAaGSW2 AaMYB16 AaJAZ8 [53] YABBY AaYABBY5 (+)AaGST formation
(+)artemisinin biosynthesisADS, CYP71AV1, DBR2, ALDH1 [54] bHLH AaGL3-like (+)AaGST formation
(+)AaTNG formation
(+)artemisinin biosynthesisAaJAZ8 [55] Phytohormones JA (+)AaGST formation
(+)AaTNG formation
(+)artemisinin biosynthesisAaHD1, AaHD8, AaJAZ8, AaMYB5 [34,41,56,57] GA (+)AaTNG formation(GA3)
(+)AaGST formation(GA1/4)
(+)artemisinin biosynthesis(GA1/4)[56,58] CK (+)AaGST formation
(+)AaTNG formation
(+)artemisinin biosynthesis[56] ABA (+)AaGST formation
(+)artemisinin biosynthesis[59] MicroRNAs miR160 (−)AaGST formation
(−)artemisinin biosynthesisAaARF1 [60] miR828 (−)AaGST formation
(−)artemisinin biosynthesisAaMYB17 [61] +, positive; −, negative. Figure 2.
Regulatory network model for trichome development in A. annua. Arrows indicate a positive influence while blocked lines indicate negative regulation.
Transcription factors
-
TFs can activate or repress the transcription of target genes by binding to specific regulatory sequences of the target genes, and play a crucial role in plant growth and development, response to adversity and secondary metabolite biosynthesis[62]. Previous studies have shown that many TF families are involved in the initiation, growth, and development of AaGSTs, with a particular focus on the R2R3-MYB, HD-ZIP, AP2/ERF, and WRKY. Additionally, because AaGSTs are closely linked to artemisinin biosynthesis, the TFs that regulate the growth and development of AaGSTs often also control the expression of key enzyme genes in the artemisinin biosynthesis pathway. These key enzymes include amorpha-4,11-diene synthase (ADS), artemisinic aldehyde double bond reductase 2 (DBR2), aldehyde dehydrogenase 1 (ALDH1), and cytochrome P450 monooxygenase (CYP71AV1), and they are identified in the AaGSTs[46].
R2R3-MYB
-
AaMYB1, AaMYB5, AaMYB16, AaMYB17, AaMIXTA1, AaTLR1, AaTLR3, and AaTAR2, belong to R2R3-MYB and are involved in regulating the growth and development of A. annua glandular trichomes.
AaMYB1 is the first R2R3-MYB TF found to actively regulate glandular trichome initiation in A. annua, and its expression is upregulated at leaf maturity and continues until flower bud formation. Studies have shown that AaMYB1 significantly activates the transcription of several key genes in the artemisinin biosynthesis pathway, especially ADS and CYP71AV1, resulting in increased artemisinin content in transgenic plants, which maybe related to the increase in the number and density of AaGST promoted by AaMYB1. Additionally, AaMYB1 can stimulate both GA4 synthesis and degradation, jointly promoting the formation of AaGSTs[40]. AaMYB16 and AaMYB5 are specific TFs of A. annua trichomes that participate in the regulation of A. annua trichome formation and their roles are reversed: AaMYB5 specifically inhibits the formation of AaGSTs without affecting the formation of AaTNGs, while AaMYB16 positively regulates AaGSTs and AaTNGs, and influences artemisinin content. Notably, AaMYB5 and AaMYB16 are unable to individually control trichome development. However, through their interactions and regulation of the binding activity of the AaHD1 promoter, they perform competing roles[41]. This study also uncovered that the expression of AaMYB5 was significantly decreased in jasmonic acid (JA)-treated A. annua, whereas the expression of AaMYB16 remained unaffected. The JA repressor, A. annua jasmonate ZIM-domain 8 (AaJAZ8), directly enhanced the repressor activity of AaMYB5 on AaHD1 transcriptional activation, while it had no impact on AaMYB16. AaMYB17, specifically expressed in AaGSTs, exerts a positive regulatory role in the initiation of AaGSTs and artemisinin synthesis while maintaining the morphology of glandular trichomes unchanged[42]. On the other hand, AaMIXTA1, which shares homology with AaMYB17 is predominantly expressed in the basal cells of AaGSTs. Its mechanism of positive regulation differs from that of AaMYB17. Through overexpression of AaMIXTA1, RNA interference, and double luciferase reporter gene detection, it has been demonstrated that AaMIXTA1 positively regulates the expression of cuticle-forming genes AaCYP77A1 and AaCYP86A1, as well as wax forming genes AaKCS5, AaCER1, and AaABCG12. This indicates that AaMIXTA1 promotes an increase in the density of AaGST and AaTNG, while positively regulating the formation of cuticle and wax[43]. The R2R3 MYB TFs TRICHOMELESS REGULATOR1 (TLR1) and TLR3 negatively regulate the development of trichomes in A. annua. In the A. annua plant, the overexpression of AaTLR1 resulted in a notable reduction of 44.7% to 64.0% in the density of AaGST compared to the wild type. Concurrently, there was a down-regulation in the mRNA levels of ADS, CYP71AV1, DBR2, and ALDH1, as well as a decrease in the artemisinin content by 11.5% to 49.4%. Conversely, AaTLR1-RNAi lines exhibited an increase in AaGST density of 33% to 93.3% above the wild type, with a concurrent up-regulation in the mRNA levels of ADS, CYP71AV1, DBR2, and ALDH1, and an increase in artemisinin content by 32.2% to 84.0%. These findings suggest that AaTLR1 is a negative regulator of glandular trichomes and artemisinin content in A. annua. Furthermore, the study revealed that AaTLR1 interacts with the LEAFY-like TF AaTLR2, facilitated by AaWOX1 (a WUSCHEL-like protein), and exerts a negative control over trichome density by suppressing gibberellin levels[44]. Research has shown that AaTLR3 plays a crucial role in glandular trichome development and stratum corneum biosynthesis. In plants with overexpressed AaTLR3, the cuticle structure of A. annua was notably disrupted, which consequently altered the morphology of its glandular trichomes. This disruption led to a reduction in the density of trichomes by 38%−42% in AaTLR3 overexpression lines when compared to the control lines. Conversely, in AaTLR3-RNAi lines, the density of trichomes saw an increase of 45%−69% relative to the control, and in gene-edited lines, this density increased by 75%−100%. Moreover, AaTLR3 also exerts regulatory control over artemisinin biosynthesis. Specifically, in A. annua with overexpressed AaTLR3, the expression of genes such as ADS, CYP71AV1, DBR2, and ALDH1, were diminished, leading to a decrease in artemisinin accumulation. Conversely, in AaTLR3-RNAi and gene-edited lines, the expression of these genes was enhanced, and the artemisinin content was significantly elevated. These findings suggest that AaTLR3 functions as a negative regulator in the development of trichomes and the biosynthesis of artemisinin in A. annua. Furthermore, AaTLR3 can regulate stratum corneum development and trichome morphology by interacting with TRICHOME AND ARTEMISININ REGULATOR 1 (AaTAR1) and CycTL (a cyclin gene, cyclin trichome less)[45]. AaTAR2 is a multifunctional TF that can affect trichome formation, artemisinin, and flavonoid synthesis in A. annua. AaTAR2 is mainly expressed in the young leaves of A. annua. Compared with the wild type, transgenic plants overexpressing AaTAR2 exhibited a substantial rise in the abundance of AaGST and enhanced expression of genes, including ADS, CYP71AV1, DBR2, and ALDH1. This upregulation corresponded with an increase in artemisinin content. In contrast, plants with inhibited AaTAR2 expression, have shown the opposite result. The morphology of AaGSTs and AaTNGs was drastically altered, with the trichome cells being crumpled and unsupported. This suggests that AaTAR2 positively regulates trichome initiation and development[46]. AaMYB108 is co-regulated by light and JA and also interacts with AaGSW2 to enhance CYP71AV1 transcription, thus promoting artemisinin biosynthesis[63]. AaMYB108-like, a homologous gene of AaMYB108 is induced by light and JA and can form a complex with AaHD8 to promote the expression of downstream AaHD1, thereby triggering the initiation of AaGSTs and promoting artemisinin biosynthesis[47].
HD-ZIP
-
Two HD-ZIP IV TFs, AaHD1, and AaHD8, regulate the initiation of trichomes in A. annua. They can act alone or jointly regulate the activity of other TFs to promote the initiation of A. annua trichomes[34,48].
AaHD1 is regulated by JA. When stimulated by exogenous JA, AaHD1 is dissociated from the inhibitory protein AaJAZ8 (a deterrent factor of the JA signaling pathway). The released AaHD1 then plays a positive role in regulating trichome initiation and artemisinin synthesis in A. annua. In the AaHD1 knockout line, the density of AaGST and AaTNG decreases significantly, leading to a significant decrease in artemisinin content[34]. AaHD8 indirectly promotes trichome initiation by controlling the expression of genes related to cuticle and wax monomer enzymes, such as AaCYP86A4 and AaFDH. Additionally, AaHD8 significantly enhances the activity of the AaHD1 promoter and positively regulates the expression of AaHD1 in the trichomes of A. annua, thus promoting the formation of AaGSTs and AaTNGs[34,48]. Moreover, AaHD8 interacts with the positive regulatory factor MIXTA-like protein AaMIXTA1 for trichome initiation and cuticle development, forming a regulatory complex and leading to enhanced transcriptional activity in regulating the expression of AaHD1 and cuticle development genes[48]. AaHD1 and AaHD8 can bind to the promoter of AaTAR2, enhancing its expression and promoting the formation of A. annua trichomes[46]. Other studies have shown that AaHD1 and AaHD8 can directly regulate AaGSW2 and promote the formation of AaGSTs[51]. Since HD-ZIP TFs usually play a regulatory role in the form of dimers, an in-depth study of the interaction mechanism of AaHD1/AaHD8 will help to reveal the specific regulatory factors regulating the formation of trichomes.
AP2/ERF
-
AaTAR1, an AP2/ERF TF, regulates the development of glandular trichomes and the biosynthesis of artemisinin in A. annua. In AaTAR1-RNAi A. annua strains, there was abnormal development of AaGSTs and AaTNGs, reduced accumulation of artemisinin, abnormal deposition of epidermal waxes, and altered cuticle permeability. Conversely, in AaTAR1 overexpressing strains, there was an increase in the expression of the two key enzyme genes of the artemisinin biosynthesis pathway, CYP71AV1 and ADS, and a significant increase in the content of artemisinin[38]. Additionally, AaTAR1 can interact with the cell cycle protein AaCycTL to regulate the biosynthesis of the stratum corneum, negatively regulate the formation of AaGSTs, and reduce artemisinin content[64]. AaWIN1 is primarily expressed in the buds, flowers, and trichomes of A. annua. It has been demonstrated that the overexpression of AaWIN1 leads to a significant increase in AaGST density and artemisinin content. Importantly, AaGSW2 is induced to be activated in AaWIN1 overexpression lines, effectively regulating the activation of AaGSTs. Furthermore, AaWIN1 interacts with AaMIXTA1 and plays a role in the biosynthesis of the cuticle layer[49]. AaSPL9 is an ERF TF, and yeast one-hybrid, dual-luciferase, and electrophoretic mobility shift assay (EMSA) have shown that AaSPL9 directly binds to the promoter of AaHD1 to activate its expression, leading to an increase in the expression of AaGSW2, which in turn regulates the initiation of AaGSTs. Overexpression of AaSPL9 increased the density of AaGST and artemisinin content, suggesting that AaSPL9 positively regulates the initiation of AaGSTs[50].
WRKY
-
WRKY is one of the plant-specific TF families. AaGSW2, a secretory glandular trichome-specific TF, which is positively regulated by the direct binding of the homeodomain proteins AaHD1 and AaHD8 to the L1-box of the AaGSW2 promoter. Overexpression of AaGSW2 significantly increased AaGST density, while AaGSW2 knockdown lines showed impaired AaGST initiation[51].
Other transcription factors
-
In addition to R2R3-MYB, HD-ZIP, AP2/ERF, and WRKY TFs, there are also other TFs involved in the development of A. annua trichomes. For example, AaSAP1 is an SAP TF, and the upregulation or downregulation of AaSAP1 transcription levels leads to an increase or decrease in AaGST density and artemisinin content, respectively, indicating that AaSAP1 positively regulates the development of AaGSTs[52]. Recent studies have revealed that AaTLR2 affects trichome development by regulating gibberellin levels[44]. In A. annua AaTLR2 overexpression lines, the trichome density was reduced, and the expression levels of ADS, CYP71AV1, DBR2, and ALDH1 also showed a tendency to be reduced, which directly led to the decrease of artemisinin content in A. annua. This shows that AaTLR2 plays a negative regulatory role in trichome initiation and artemisinin biosynthesis in A. annua. The study revealed a MADS-box gene AaSEPALLATA1 (AaSEP1), which can be induced by JA and light signals and promotes AaGST initiation in A. annua[53]. Overexpression of AaSEP1 in A. annua significantly increased AaGST density and artemisinin content. This study found that AaSEP1 enhances the activation of the downstream AaGST promoter gene AaGSW2 by interacting with AaMYB16. In addition, the interaction between AaSEP1 and AaJAZ8 is an important factor in JA-mediated AaGST initiation. The study also found that AaSEP1 interacts with light morphogenetic protein 1 (AaCOP1), the main inhibitor of light signaling. The YABBY gene family, comprising a modest array of TFs within seed plants is pivotal in orchestrating the growth and development of plant leaves[65]. AaYABBY5, a distinguished family member, was cloned and characterized in a recent study. The research revealed that AaYABBY5 directly binds to the promoters of CYP71AV1 and DBR2 or indirectly regulate ADS and ALDH1, thereby activating their gene expression and substantially enhancing the concentration of artemisinin[54]. Furthermore, the study demonstrated that the trichome density in plants overexpressing AaYABBY5 was significantly elevated compared to control plants, while antisense plants exhibited a marked reduction in trichome density[66]. These findings underscore AaYABBY5's role as a positive regulator in the biosynthesis of artemisinin and the development of trichomes. Some scholars have successfully isolated a homolog of AtGL3 (GLABRA3) from A. annua, named AaGL3-like, which is categorized in the bHLH TF family. In-depth studies showed that AaGL3-like was able to be induced by JA and significantly increase the final accumulation of artemisinin content by regulating the density of trichomes in A. annua[55]. A study has shown that the increase in the expression level of Transparent testa glabra 1 (TTG1) is related to the increase in glandular trichome density and artemisinin production in A. annua leaves[67].
These TFs are involved in regulating genes associated with cell division, growth, and differentiation. They exert their effects by activating or inhibiting specific gene expression to control trichome formation and development in A. annua. A comprehensive understanding of the functions and regulatory mechanisms of these TFs will contribute to elucidating the molecular mechanisms underlying trichome growth and development in A. annua. It may offer novel strategies for cultivating artemisinin high-yield varieties.
Phytohormones
-
Phytohormones are signaling molecules that regulate specific cellular processes and play a crucial role in plant growth, development, and environmental stress responses[68]. Currently, research has found that phytohormones such as jasmonic acid (JA), gibberellins (GAs), cytokinin (CK), and abscisic acid (ABA) regulate the growth and development of A. annua trichomes by inducing TFs and some of them affect the biosynthesis of artemisinin.
Jasmonic acid
-
JA belongs to the lipid derivatives and is a plant-secondary metabolism stimulator[69]. Since artemisinin content is closely related to the density of AaGST, JA can regulate the growth and development of AaGSTs by affecting genes related to the development of AaGSTs. For example, JA induced the specific expression of trichome-specific fatty acyl-CoA reductase 1 (TFAR1) in AaGSTs, which resulted in a significant increase in the density and size of AaGSTs on A. annua leaves and also promoted the biosynthesis of artemisinin[56]. When AaAOC, a key enzyme in the JA synthesis pathway is overexpressed in A. annua, the endogenous JA content in A. annua increases, which leads to an increase in the density of AaGST[57]. JA also induces the formation of AaGSTs with the help of the HD-ZIP family of TFs, AaHD1 and AaHD8. JA regulates the function of the AaHD1 gene at both the transcriptional level and the protein level, thereby positively regulating the early development of secretory glandular trichomes on the leaf surface of A. annua; the expression of AaHD1 was significantly increased in JA-treated A. annua plants, which promotes the formation of AaGSTs and AaTNGs[34]. In addition, JA treatment of A. annua plants significantly downregulated the expression of AaMYB5, which acts with AaJAZ8 to regulate the formation of AaGSTs[41].
Gibberellins
-
GAs are diterpenoids, which are present in all higher plants as a class of phytohormones and play a role in many developmental processes. It has been shown that the density of AaTNG on A. annua leaves increased significantly after GA3 treatment, whereas there was no significant change in the density of AaGST and artemisinin content[56], and it has also been recently shown that GA3 does not stimulate the formation of AaGSTs or the biosynthesis of artemisinin, whereas endogenous GA1/GA4 can promote AaGSTs formation and artemisinin synthesis by AaMIXTA1, which promotes AaGSTs formation and artemisinin synthesis[58], which may be because exogenously applied GA3 is not the active form for AaGSTs formation.
Cytokinin
-
CK can promote the development of trichomes in A. annua, and 6-benzylaminopurine (BAP) was found to increase the density of AaTNGs and stimulate the formation of AaGSTs. The density of glandular trichomes in BAP-treated plants was significantly increased and the glandular trichomes were enlarged, but BAP did not stimulate artemisinin biosynthesis[56].
Abscisic acid
-
ABA is a natural plant growth regulator that can promote coordinated plant growth and improve plant growth quality. Research has found that externally applied ABA can reverse the negative effects of copper treatment, increase the density and size of AaGSTs under copper stress, and increase the content of artemisinin under copper stress[59].
MicroRNAs
-
Like TFs, microRNAs (miRNAs) are also indispensable regulatory factors in trichomes. MiRNAs are non-coding RNAs found in eukaryotes with endogenous regulatory functions. They are approximately 20−25 nucleotides in size and play a crucial role in plant development and secondary metabolism through different sequence-specific interaction patterns with targets. Studies have shown that miR160 reduces the formation of AaGSTs by targeting and cleaving AaARF1. The results showed that in the miR160-overexpressing strains, the density of AaGST and artemisinin content decreased significantly. On the contrary, in the miR160 knockout strains, the density of AaGST increased compared with the wild type, and the content of artemisinin was also significantly increased[60]. Recently, miR828 was found to degrade AaMYB17 in A. annua plants. Overexpression of miR828 reduced the density of AaGST by about 60%, and artemisinin content was consequently reduced[61]. The study of miRNA provides new possibilities for increasing artemisinin content, but further in-depth research is needed on this regulation.
Others
-
Under natural conditions, plants are always exposed to environmental stresses. In addition to TFs and phytohormones, some adversity factors, such as drought, salt, heavy metals, and other abiotic stresses, also have some effects on the formation and development of trichomes in A. annua.
It has been discovered that mild-to-moderate drought stress results in a reduction in both the density and volume of AaGSTs, in comparison to non-stress conditions[70]. This decrease in artemisinin content was found to be closely associated with the reduction. Under 50 and 100 mM salt treatments, the length and width of AaGSTs increased, along with an increase in the number of AaGSTs. However, under 200 mM salt stress, the volume of AaGSTs decreased, while the density of AaGST remained constant throughout all stages of plant life[37]. Moreover, increasing copper concentration led to a decrease in the density, average width, and length of AaGSTs, as well as a decline in artemisinin content in A. annua plants exposed to copper stress when compared to the control group[59]. Arsenic treatment (0~45 mg/kg) of A. annua resulted in an increase in artemisinin content, which may be related to the expansion of artemisinin-producing apical cells on the AaGSTs after Arsenic treatment[71]. High cadmium stress (20, 40 mg/kg) had a significant negative effect on the length, width, and density of the AaGST, as well as the artemisinin content, which was all significantly negatively affected, and the higher the cadmium concentration, the greater the effect[72]. Exogenous supplementation of strigolactone (GR24) under cadmium stress or spraying NO on the stressed plants could significantly improve the situation[72,73]. In a recent study, the carbon nanomaterial graphene was found to block the biogenesis of miRNAs and directly disrupt the function of the miR828-AaMYB17 module, which enhanced the initiation of AaGSTs and induced the production of artemisinin[61].
Although many studies have reported the effects of non-biological stress signals on the trichomes and artemisinin content of A. annua, there is relatively little research on the effects of biological stress signals on the trichomes and artemisinin content of A. annua. Scholars have found that A. annua plants inoculated with Glomus macrocarpum and Glomus fasciculatum have a higher density of AaGSTs on their leaves compared to non-fungal root plants, and the detected artemisinin content is also higher. There is a strong positive linear correlation between the density of AaGST on the leaves and artemisinin concentration[74].
-
Plant secondary metabolites are a class of biomolecules synthesized by secondary metabolic pathways, which are important protective barriers for plants to adapt to the environment and cope with adverse environments. Artemisinin is a sesquiterpenoid synthesized and stored in AaGSTs, and its biosynthetic pathway belongs to the isopentenyl-like metabolic synthesis pathway originating from isopentenyl diphosphate (IPP) and dimethylallyl diphosphate (DMAPP)[75,76] (Fig. 3). The mevalonate acid (MVA) pathway significantly influences the synthesis of farnesyl pyrophosphate (FPP), with its inhibition leading to an 80.4% reduction in artemisinin content. Conversely, disruption of the methylerythritol phosphate (MEP) pathway results in a mere 14.2% decrease in artemisinin content[77]. In the course of FPP synthesis, a molecule of IPP derived from the MEP pathway is initially coupled with a molecule of DMAPP produced by the MVA pathway to form geranyl diphosphate (GPP). Subsequently, this GPP is transported to the parietal cells and the cytoplasm, where it is converted into the universal precursor FPP by the enzyme farnesyl diphosphate synthase (FPS), utilizing an additional molecule of IPP originating from the MVA pathway[78,79]. Sesquiterpenoids are synthesized using FPP as a substrate and catalyzed by sesquiterpene enzymes. ADS is a specific sesquiterpene enzyme for artemisinin synthesis, and FPP is catalyzed by ADS to produce amorpha-4,11-diene (AD)[80−83], and AD is converted to artemisinic alcohol (AAOH) via CYP71AV1[84,85]. Subsequently, AAOH is oxidized to artemisinic aldehyde (AAA) by alcohol dehydrogenase 1 (ADH1) and CYP71AV1[84,85]. It is worth noting that artemisinin aldehyde can undergo catalysis by various enzymes, leading to the production of different metabolites. Specifically, the catalysis of AAA by CYP71AV1 and ALDH1[86] results in the formation of artemisinic acid (AA), which eventually leads to the synthesis of arteannuin B (AB). Alternatively, AAA can be catalyzed by DBR2 and then ALDH1, resulting in the production of dihydroartemisinic aldehyde (DHAAA)[87], dihydroartemisinic acid (DHAA)[86] and ultimately artemisinin. AA and DHAA undergo non-enzymatic photo-oxidation to ultimately produce artemisinin B and artemisinin, respectively[88,89]. In addition, AAOH can generate other intermediates involved in artemisinin synthesis. For instance, AAOH can undergo catalysis to yield dihydroartemisinic alcohol (DHAAOH)[90]. Subsequently, DHAAOH can be catalyzed by CYP71AV1 and ALDH1 to form dihydroartemisinic aldehyde (DHAAA). Additionally, DHAAA can be specifically reduced back to DHAAOH by the enzyme dihydroartemisinic aldehyde reductase 1 (RED1), allowing it to continue its involvement in the synthesis of artemisinin[91].
Figure 3.
Biosynthetic pathways of artemisinin biosynthesis. AA, Artemisinic acid; AAA, Artemisinic aldehyde; AAOH, Artemisinic alcohol; AB, Arteannuin B; AD, amorpha-4,11-diene; ADH1, alcohol dehydrogenase 1; ADS, amorpha-4,11-diene synthase; ALDH1, aldehyde dehydrogenase 1; CPR, cytochrome P450 reductase; CYP71AV1, cytochrome P450 monooxygenase; DBR2, artemisinic aldehyde double bond reductase 2; DHAA, dihydroartemisinic acid; DHAAA, dihydroartemisinic aldehyde; DHAAOH, dihydroartemisinic alcohol; DMAPP, dimethylallyl diphosphate; FPP, farnesyl diphosphate; FPS, farnesyl pyrophosphate synthase; GPP, geranyl diphosphate; IDI, isopentenyl-diphosphate isomerase; IPP, isopentenyl diphosphate; MEP, 2-C-methyl-D-erythritol 4-phosphate; MVA, mevalonic acid; RED1, dihydroartemisinic aldehyde reductase 1.
-
Artemisinin is primarily synthesized, secreted, accumulated, and stored in the secretory trichomes of A. annua[92]. Hence, the formation and development of secretory trichomes are crucial for increasing artemisinin yield. The biosynthesis of artemisinin is divided into upstream and downstream pathways, with the upstream pathway being relatively clear, while the downstream steps are not yet fully elucidated. Therefore, improving artemisinin yield by focusing on key enzymes in the metabolic pathway is limited. In contrast, as the 'chemical factory' of artemisinin biosynthesis, trichomes contain all the necessary factors for artemisinin biosynthesis and have unparalleled advantages in increasing artemisinin content in A. annua. Regulating trichomes can bypass the uncertainties and controversies in the artemisinin biosynthesis pathway, allowing for more efficient macro-control of artemisinin biosynthesis[6].
In recent years, scientists have explored factors affecting the formation and development of A. annua trichomes, including transcription factors, plant hormones, and microRNAs. These studies reveal the key role of trichomes in artemisinin biosynthesis and provide an important theoretical basis for subsequent research. With the continuous development of theories and technologies such as molecular biology, cell biology, multi-omics, molecular breeding, gene editing, and single-cell transcriptome sequencing (scRNA-Seq), more and more research methods are being applied to the study of A. annua trichome development and artemisinin biosynthesis. For example, gene knockout, silencing, and gene editing can construct mutants with target gene deletions, and then observe the differences between mutants and wild types to verify the impact of target gene function on artemisinin synthesis or trichome development. In addition, transcriptomics and metabolomics analysis facilitate the study of trichomes and artemisinin biosynthesis. After screening and identifying target genes for molecular breeding, new artemisinin high-yield varieties of A. annua can be cultivated using a combination of traditional breeding and molecular breeding. These methods all provide important ideas for improving artemisinin yield. ScRNA-Seq is a high-throughput genome sequencing technology that has tremendous advantages in identifying intercellular heterogeneity, analyzing gene expression at high cellular resolution, and constructing regulatory networks of TFs[93]. In the last few years, scRNA-Seq technology has developed rapidly in the field of plant research. For example, a study has revealed the early developmental dynamics of leaf vein cells in Arabidopsis cotyledons using scRNA-Seq technology, which provides new cell-type specific gene expression profiles and potential marker genes for future studies on the development of plant vascular tissues[94]. In addition, scRNA-Seq technology has been applied to the medicinal plant Nepeta tenuifolia L., where multiple differentially expressed genes that may regulate the growth and development of glandular trichomes in Nepeta tenuifolia were identified, providing a basis for exploring the development and differentiation of plant cells, especially the initiation and development of glandular trichomes[95]. This method can be similarly applied to the study of A. annua, which will greatly facilitate the discovery of important functional genes, deepen the understanding of the complex molecular mechanisms of glandular trichome formation and development, artemisinin biosynthesis and their regulatory networks, and provide more comprehensive scientific guidance for the improvement of A. annua varieties and the enhancement of artemisinin production.
Regarding the formation and development of trichomes in A. annua, although transcription factor families such as AP2/ERF, WRKY, MYB, and HD-ZIP have been shown to play important roles in regulating trichome development, the vast number of plant transcription factors and the complex regulatory network means that the underlying molecular mechanisms are mostly unknown. Therefore, further exploration of the molecular mechanisms of trichome development is necessary, especially the synergistic regulatory effects between transcription factors and miRNAs. This will help us better understand the trichome development process and provide a theoretical basis for improving trichome characteristics through genetic engineering. Previous studies have confirmed that changing trichome characteristics, such as regulating trichome morphology with TAR1[38] and regulating trichome density with TAR2[46], can significantly affect artemisinin yield. In addition, based on a thorough understanding of the artemisinin biosynthesis pathway, reorienting metabolic flow through regulation to increase artemisinin yield will also be a key research direction we can focus on.
In summary, as the factory of artemisinin biosynthesis, AaGST plays a significant role in improving artemisinin yield. The AaGST is a typical secretory glandular trichome, which is not present in the model plants Arabidopsis and rice. Therefore, AaGST can be studied as a model organ for secretory glandular trichomes and can be used as a reference for other species.
-
The authors confirm contribution to the paper as follows: conceptualization and supervision: Tan H; draft manuscript and figure preparation: Zhao Q; manuscript review and editing: Li M, Zhang M. All authors reviewed the results and approved the final version of the manuscript.
-
Data sharing not applicable to this article as no datasets were generated or analyzed during the current study.
This work was supported by grants from the National Natural Science Foundation of China (82122072), the Natural Science Foundation of Shanghai Municipality (21ZR1477800), and the Traditional Chinese Medicine Research Project of Shanghai (2024PT010). The authors declare that they have no competing interests.
-
The authors declare that they have no conflict of interest. Hexin Tan is the Editorial Board member of Medicinal Plant Biology who was blinded from reviewing or making decisions on the manuscript. The article was subject to the journal's standard procedures, with peer-review handled independently of this author and the research groups.
-
# Authors contributed equally: Qiaojuan Zhao, Mingyu Li, Minghui Zhang
- Copyright: © 2024 by the author(s). Published by Maximum Academic Press, Fayetteville, GA. This article is an open access article distributed under Creative Commons Attribution License (CC BY 4.0), visit https://creativecommons.org/licenses/by/4.0/.
-
About this article
Cite this article
Zhao Q, Li M, Zhang M, Tan H. 2024. Glandular trichomes: the factory of artemisinin biosynthesis. Medicinal Plant Biology 3: e019 doi: 10.48130/mpb-0024-0018
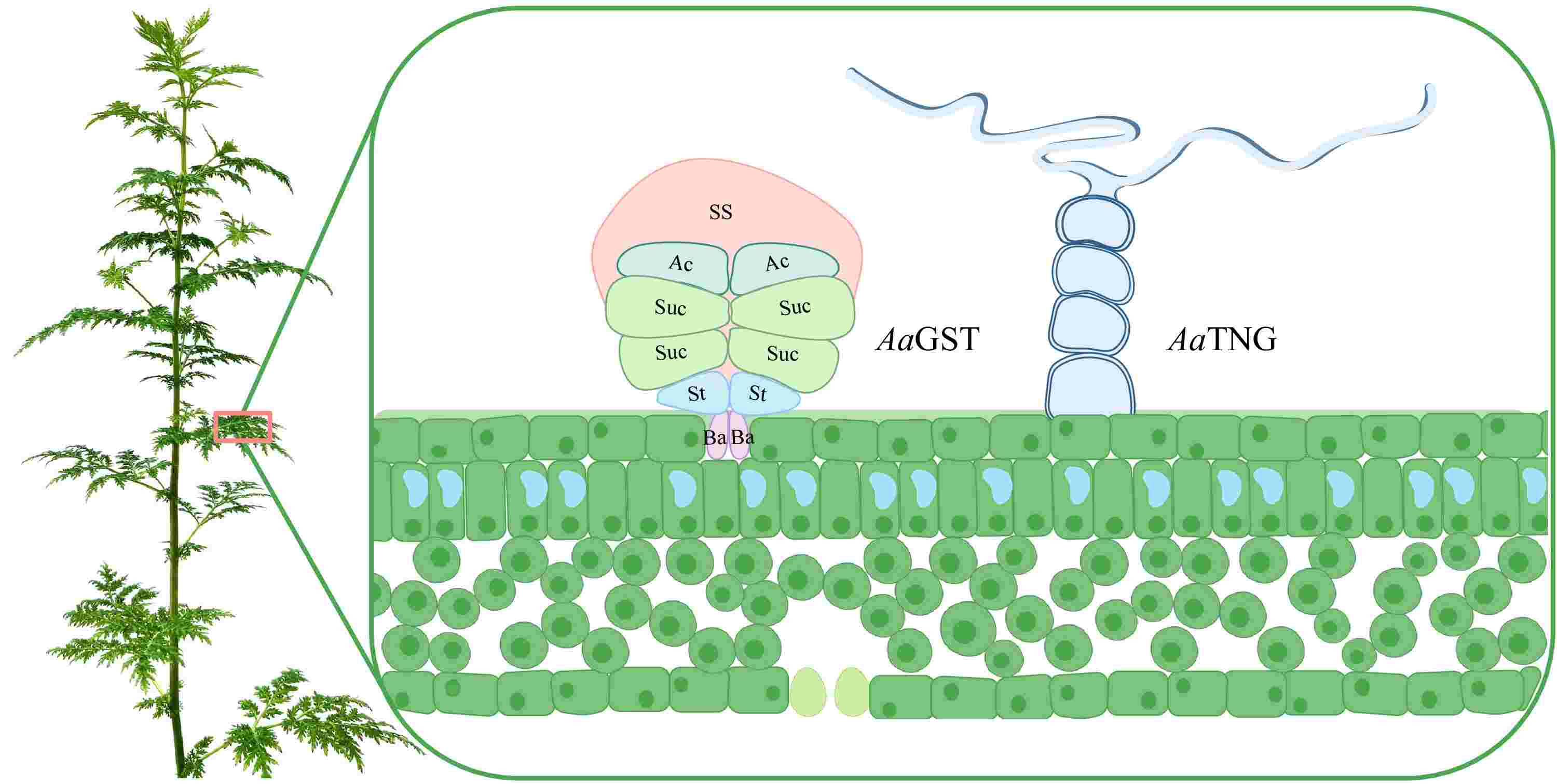