-
Due to major changes in natural conditions and human influence, salinity is one of the most serious and significant hazards to agricultural land. Salts at lesser concentrations inhibit growth, whilst salts in larger concentrations might cause the plant to die. High pH, sodium (Na+) adsorption ratio, exchangeable sodium percentage, and electrical conductivity are all characteristics of saline soils. Large amounts of Na+ and Cl‒ ions taken in by plants disrupt plant physiology by altering metabolic processes[1,2]. Increasing salinity causes arable agricultural land to be converted into unproductive wastelands. Significant amounts of irrigated land around the world are affected by salt, which has an impact on the average yields of main food crops[3]. Plants' physiological processes are hampered by high-salinity soils. The cumulative influence of salinity on numerous physiological and biochemical properties such as mineral ion homeostasis, water balance, osmolyte accumulation, antioxidant metabolism, nitrogen fixation, and photosynthetic capacity of plants causes changes in plant growth and development[4]. Respiration, photosynthesis, nitrogen fixation, residue decomposition, nitrification, denitrification, soil biodiversity, and microbial activity are all harmed by high salt levels, resulting in a decrease in farm productivity and yield[5]. However, due to their normal salt tolerance profile, these crops have a limited global reach and cannot be used in soils with moderate or high electrical conductivity (EC) levels[6,7]. Morton et al. also stated that despite the research community's efforts, only a few salt tolerance genes have been identified with real applications in improving saline soil productivity[7].
High salt concentrations (NaCl) not only increased stress ethylene production, but also caused ion toxicity, oxidative stress, and osmotic potential disruption in plants[2]. It has been shown that when plants are exposed to salt, their ethylene levels rise, altering the physiological processes of the plant, and resulting in the inhibition of plant development and maybe even plant death[8,9]. The abnormalities in various essential cellular biochemical pathways/reactions that work in cellular organelles including chloroplast and mitochondria are caused by salt stress (SS)-induced generation of reactive oxygen species (ROS)[1,10−13]. Plant cells have a well-developed antioxidant defense system to prevent ROS-induced damage by regulating the cell's redox state, which aids in the scavenging of ROS. Every organelle in plant cells has one or more antioxidants that act on a specific ROS to detoxify it[2,14,15].
Any external element that harms plant growth, productivity, reproductive potential, or survival is referred to as stress in plants. Abiotic stressors are negative environmental factors that affect soil microbial diversity and physicochemical properties, resulting in significant yield loss[2,16,17]. Abiotic stressors have a negative impact on plant development and growth in general. High salinity is anticipated to harm approximately half of all agricultural land on the planet, according to estimates[18]. The removal of salt from saline soil is a time-consuming and expensive procedure[19]. However, physical and chemical procedures have been used to reclaim saline soils for a long time. Scraping, flushing, and leaching procedures are used in the physical process to remove soluble salts from the root zone[20]. However, gypsum and lime are commonly used as neutralizing agents in chemical techniques[21]. Yet, these methods are not only unsustainable, but they are also ineffective when the salt content is too high. According to Vejan et al. microorganisms that have the potential to promote plant development may be very useful instruments for ensuring sustainable agriculture[22]. As a result, PGPR has received a lot of interest in recent decades as a potential long-term solution for increasing agricultural output and stress tolerance. Over the past 20 years, there has been a significant gain in information about PGPRs and their mechanisms of action[23].
Plant growth-promoting rhizobacteria (PGPR), a group of beneficial bacteria found in the rhizosphere and rhizoplane of plants, are the most environmentally friendly and superior alternative to synthetic agrochemicals and other conventional agricultural practices in enhancing plant growth and SS tolerance, as well as in achieving sustainable agriculture[2]. Though they also include certain inherent ambiguities that require careful elucidation, they have significant implications for sustainable agriculture. The bacteria that can tolerate a high range of salinity are known as ST-PGPR. To properly utilize PGPR, one must have a solid grasp of the complex connections that exist between microbes, plants, and the environment[24]. Sustainable agriculture is at the forefront of global efforts to address environmental preservation and food security. In this context, PGPR has emerged as potentially revolutionary for sustainable agricultural practices[25]. These beneficial bacteria coexist with plants in the rhizosphere, assisting them in absorbing nutrients, fending off disease, and withstanding SS[26]. Its ability to enhance plant development and minimize stress is attributed to PGPR's principal function in activating plant signaling pathways[24].
Numerous PGPRs have an enzyme called 1-aminocyclopropane-1-carboxylate deaminase ACCD, which may breakdown ACC into ammonia and α-ketobutyrate, lowering the amount of ethylene in the plants[2,27]. As a result, the PGPR-containing ACCD can reduce ethylene synthesis and its negative effects on plants caused by abiotic stress. In recent years, research has shown that using ST-PGPR in saline agriculture can boost productivity while also improving soil fertility[28,29]. Their ability to create osmoprotectants, suitable solutes, and specialized transporters is linked to their adaptive responses to SS. ST-PGPR is presently employed as a bioinoculant to improve crop yields, defend against phytopathogens, and improve soil health. The current study focuses on the important role of ST-PGPR in increasing crop productivity in salt provisional-affected soil. Apart from that, ST-PGPR-mediated mechanisms, as well as novel insights and prospects for crop productivity enhancement in salinity-stressed environments, are explored.
-
Any external element that harms plant growth, productivity, reproductive potential, or survival is referred to as stress in plants. Abiotic stressors are negative environmental factors that affect soil microbial diversity and physicochemical properties, resulting in significant yield loss[2,16]. Constant human activities, such as intense agricultural techniques aiming at increasing agricultural production or degradation of arable lands due to industrialization, are the main cause of the abrupt increase in abiotic stresses[30,31]. Salinization of arable land is thought to be the most dangerous abiotic factor for agricultural production. Soil salinization is one of the nine main risks to soil functions that have been recognized in the most recent report 'The Status of the World's Soil Resources' by the Food and Agriculture Organisation[32]. Global soil salinization is increasing, and in recent years, this rapid process has also led to food shortages in several nations. Food security in the delta areas of Bangladesh, India, and Myanmar—which produce a majority of the world's rice—is seriously threatened by the salinization of coastal soil[33,34]. As estimated by Kumar & Sharma the irrigated salt-affected areas cost USD
12 billion in yearly revenue losses worldwide[18].$ In India, 6.74 million hectares are plagued by salinity. An estimated 10% more land is expected to get salinized annually by 2050 when almost half of all arable land will have been damaged by salt. Twelve states and one Union Territory have 44% saline soils, and 11 states have 47% sodic soils[18]. Changes in soil physicochemical qualities lead to irreversible land degradation in salt-stressed areas, resulting in a massive reduction in world food output. Furthermore, prolonged SS causes significant changes in plant physiological and morphological characteristics, culminating in nutritional cytotoxicity and osmotic imbalances[1,2,10]. It has an impact on nearly all aspects of plant development, including germination, vegetative growth, reproductive development, and photosynthesis (Fig. 1).
Photosynthesis rate is lowered under SS due to a fall in water potential, resulting in a significant reduction in the supply of carbohydrates essential for cell development[2]. When large concentrations of Na+ and Cl− accumulate in chloroplasts, photosynthesis is hindered, resulting in lower chlorophyll content in plants. Reduced photosynthesis rates can also be caused by declines in photosystem II (PSII) activity, electron transport system (ETS), photophosphorylation activity, photosynthetic enzymes, chlorophylls, and carotenoid content, in addition to the aforementioned reasons[35]. Additionally, SS disrupts photorespiration, physiological and metabolic activities, thereby interrupting the homeostasis of the normal cell[36].
-
Root performance is determined by the root architecture of the plant. A branching root system allows plants to quickly obtain water and nutrients from the soil, increasing the rate of plant water loss substitution[37]. A well-developed root system is required for deep penetration into the soil layers and quicker water and nutrient uptake[38]. Plants adapt their root architecture to the surrounding environment when they are exposed to SS. Salt stress causes the root cortex to shrink to minimize the distance between the epidermis and the stele, allowing for the simple uptake of important minerals while inhibiting undesirable ones[39]. It frequently stimulates hypodermis and endodermis suberization in woody tree roots, resulting in the production of the Casparian strip (a specifically modified carbohydrate cell wall found in the apoplastic space of a plant cell) closer to the root apex. The Casparian strip, together with the endodermis and exodermis, forms a tight hydrophobic barrier in halophytes that regulates the apoplastic pathway and maintains the flow of solutes into the cytoplasm through the selectively permeable plasma membrane. Furthermore, during SS, halophytes have a thick cytoplasm in the meristematic zone of the root tips, which functions as a barrier against unwanted influx of solutes into the plant cell[40]. Furthermore, they have a thicker primary root that works as a sink for Na+ ion sequestration, shielding the plants from SS by preventing Na+ buildup in the lateral roots and young leaves[41]. However, describing a general scenario of root reactions to external stimuli is difficult since it differs from species to species and between different root growth stages. Finally, the roots' remarkable ability to adjust to varied external stress situations is due to their significant root flexibility[42,43]. By altering the amounts of hormone synthesis, distribution, and signal transduction, plants may control and coordinate their growth and SS tolerance, promoting survival or protection from environmental stress[2,10].
-
Salt tolerant-plant growth promoting Rhizobacteria (ST-PGPR) are soil-borne bacteria that are connected with plants and have the potential to promote plant development through a variety of ways. Kloepper classified PGPR based on their intrinsic characteristics, such as (i) root colonization, (ii) plant growth promotion, and (iii) the ability to adapt, live, proliferate, and compete with other microbial flora to exhibit their plant growth promotion and protection activities[44]. Additionally, PGPR may categorize objects based on their functional qualities. According to reports, there are two ways in which PGPRs promote plant development[45]. ST-PGPRs primarily activate the plant's metabolic pathways, which include enhancing the storage of nutrients in plants, catalyzing the synthesis of growth hormones, and promoting the formation of siderophores and antioxidants. Additionally, ST-PGPRs are in charge of producing a variety of phytohormones, such as auxins and cytokinins, which aid in the growth of roots and shoots and refine nutrient supplementation through mineral solubilization, N2 fixation, and others[29].
Plants can be stunted by a variety of stressors, such as salinity stress (SS), drought stress (DS), heavy metal stress (HMs), floods, diseases, high temperatures (HT), and others that may be made worse by human activity on top of natural pressures. ST-PGPR can lessen these stresses by using numerous techniques, including siderophore synthesis, N-fixation, PO43− solubilization, and ACCD production[16]. In salt-affected soil, Peng et al. chose a consortium of three bacterial strains (Streptomyces sp. X52, Pseudomonas sp. P8, Peribacillus sp. P10) and injected the roots of maize plants[46]. The consortium's capacity to withstand SS was shown to accelerate plant development. To preserve their cytoplasmic osmolarity when exposed to SS, the majority of rhizospheric bacteria release osmoprotectants, such as glutamate, proline betaine, ectoine, proline, and trehalose[47]. Separate research by Dong et al. claims that P. aeruginosa HG28-5 improves plant SS tolerance by controlling ABA signaling and the Na+/K+ balance. This increases antioxidant activity and reduces SS in the plant[48].
Various forms of PGPR
-
Extracellular plant growth-promoting rhizobacteria (ePGPR) and intracellular plant growth-promoting rhizobacteria (iPGPR) are the two basic types of PGPR[49]. The ePGPR lives in the rhizosphere (on the rhizoplane) or the gaps between the cells of the root cortex, whereas iPGPR lives mostly inside the root cell's specialized nodular structures. Azotobacter, Serratia, Azospirillum, Bacillus, Caulobacter, Chromobacterium, Agrobacterium, Erwinia, Flavobacterium, Arthrobacter, Micrococcus, Pseudomonas, and Burkholderia are among the bacterial genera covered in ePGPR. Allorhizobium, Bradyrhizobium, Mesorhizobium, and Rhizobium, as well as Frankia species, are endophytic microorganisms that can fix atmospheric nitrogen, especially for higher plants[50,51].
ST-PGPR's function as a plant growth enhancer
-
ST-PGPR promotes plant growth in a variety of ways, including (i) biofertilizers (which increase soil nutrient availability), (ii) phytostimulators (which stimulate plant growth by producing phytohormones), (iii) rhizoremediators (which modulate pollutant availability through metal solubilization), and (iv) bio-pesticides (controlling plant pathogens and diseases by the secretion of lytic enzymes and metabolic compounds)[52]. ST-PGPR strains can boost plant growth through numerous methods, both particular and non-specific. ST-PGPR strains stimulate plant development by a variety of methods, including improving soil nutrient availability, promoting root growth, cell division, elongation, and metabolic stability. However, ST-PGPR strains improve plant development by non-specific mechanisms such as reducing HM-induced toxicity and plant infections, as well as enhancing systemic resistance induction. The next sections go over the ST-PGPR strains detailed plant growth-promoting processes (Fig. 2).
Figure 2.
Plant growth promotion activities of stresses tolerant PGPRs to enhanced the plant efficiency. HMs: Heavy metals; IAA: Indole-3 Acetic acid; ABA: Abscisic acid; SOD: Superoxide dismutase; POD: Peroxidases; APX: Ascorbate peroxidase; CAT: Catalase; GPX: Glutathione peroxidase; VOCs: Volatile organic compounds; EPS: Exopolysaccharides.
Plant growth-promoting substances and metabolites such as phytohormones, siderophores, and ammonia are produced by ST-PGPR strains, which enhance soil nutrient availability through PO43‒ solubilization, N-fixation, ACCD, promoting antioxidant enzymes and organic compound mineralization thereby encouraging plant development[6,8]. Plant roots exude a variety of substances, including proteins, carbohydrates, sugars, mucilage, organic acids, and carbohydrates[53].
ST-PGPR-induced salt stress tolerance
-
On a global scale, salinization is the most devastating environmental concern, affecting a billion hectares of land[54,55]. Continuous osmotic stress (OS) affects plant reproductive processes, causing delayed emergence of new leaves as well as a reduction in flowering and fruiting rates[56]. It is now commonly known that ST-PGPR has the potential to cope with high salt concentrations in the soil by nature. According to Gupta et al. osmotic accumulation, hydraulic conductance, preserving the greater osmotic conductance, photosynthetic activities, and sequestering harmful Na+ ions are likely the mechanisms behind this tolerance[10]. According to Nakbanpote et al. Serratia sp. and Pseudomonas sp. have unique abilities such as producing IAA, fixing nitrogen, and solubilizing phosphate in 8% saline conditions. Pseudomonas sp. was also evaluated for better germination of Oryza sativa L[57]. According to a recent study by Ghosh et al. rhizobacteria may help plants experience less stress[58]. The application of microbial inoculants can increase plant health in salinized soils via efflux systems[59]. These microbial inoculants create IAA, gibberellins, and other phytohormones in plants, which assist regulate plant nutrient levels and balance ion influx in plants under SS[1,10]. Their use in the form of bio-inoculants/bio-formulations boosts agricultural productivity while also assisting plant survival in extremely salty environments (Table 1).
Table 1. Salt-tolerant plant growth-promoting rhizobacteria and their potential involvement in increasing yield and growth in a variety of plants and crops.
ST-PGPR Mode of action Crops Ref. B. subtilis SU-12, Bradyrhizobium japonicum, and Serratia proteamaculans Antioxidant enzyme and Proline content Glycine max [60] K. oxytoca Rs-5, Bacillus sp. SL-44, Bacillus
sp. SL-14, and Bacillus sp. SL-13Antioxidant enzymes and photosynthetic pigment Gossypium hirsutum [61] P. pseudoalcaligens Siderophore, PO43‒ solubilization, and IAA production Cicer arietinum [62] Azotobacter chroococcum Improved K+/Na+ ratio, proline concentration, and polyphenol content Zea mays [63] Pseudomonas and Rhizobium sp. Water use efficiency, chlorophyll content, and photosynthetic rate Vigna radiata [64] Enterobacter cloacae N-fixation, Production of growth-promoting compounds Various vegetables and crops [65] P. aeruginosa EPS production Helianthus annuus [66] Klebsiella, Ochrobactrum, Pseudomonas,
and AgrobacteriumIAA production, PO43− solubilization, ACCD Arachis hypogaea [67] B. subtilis Nitrogen fixation PO43− solubilization, Production of plant hormones, Biocontrol of plant pathogens Most plants, including vegetables, cereals, and fruits [68] Rhizobium sp. ACCD, IAA production, and PO43‒ solubilization Brassica napus [69] Halobacillus sp. GSP 34 and
H. dabanensis SB-26N-fixation and IAA production O. sativa [70] P. aeruginosa GI-1, and
Burkholderia gladioli GI-6N assimilation, PO43‒ solubilization, IAA production, siderophores generation, and CAT activity Triticum aestivum L. [71,72] Bacillus and Pseudomonas sp. N-fixation and IAA production Pisum sativum L. [16] Bacillus sp. IAA production, PO43‒ solubilization, ACC deaminase, and HCN production P. sativum L. [2] B. cereus and B. marisflavi IAA production, PO43‒ solubilization, ACCD activity, Biofilm formation, HCN, and Siderophore production P. sativum and Z. mays [73] Arthrobacter sp., P. plecoglossicida,
Kocuria rosea, and Bacillus isolatesIAA production, Zn and PO43‒ solubilization, NH3 and siderophore production P. sativum [74] Enterobacter and Pseudomonas sp. ACCD activity O. sativa [75] P. aeruginosa HG28-5 Increased morphological and physiological parameters like elevating the antioxidants enzyme level, reducing oxidative stress, and up-regulating ABA pathway genes Solanum lycopersicum [48] Burkholderia sp. BK01 Enhanced enzymatic activities like SOD, POD, and CAT Arabidopsis thaliana [76] B. velezensis CC03 and
P. nicosulfuronedens AP01PO43‒ solubilization and pathogen suppression thereby increasing the RWC, PRP accumulation Hibiscus sabdariffa [77] N: Nitrogen; IAA: Indole-3-acetic acid; ACCD: 1-aminocyclopropane-1-carboxylate deaminase; P: Phosphate; HCN: Hydrogen cyanide; EPS: Exopolysaccharide; K+: Potassium; Na+: Sodium; Zn: Zinc; CAT: Catalase; ABA: Abscisic acid; SOD: Superoxide dismutase; POD: Peroxidase; RWC: Relative water content; PRP: Proline-rich protein. These helpful microbes for plants that can withstand SS and play a significant role in agriculture. Niu et al. have demonstrated their ability to increase agricultural yield in dry and semiarid areas[78]. The genera Agrobacterium, Bacillus, Klebsiella, Pseudomonas, Enterobacter, Streptomyces, and Ochromobacter have the greatest track records for boosting agricultural productivity in salinized environments[45,67,79]. Further Gupta et al. also found the beneficial effect of Bacillus and Pseudomonas sp. in ameliorating the SS[2]. The diazotrophic ST-bacterial strains of Agrobacterium, Pseudomonas, Ochrobactrum, and Klebsiella that were isolated from the roots of Arthrocnemum indicum, a halophytic plant, demonstrated SS tolerance ranging from 4% to 8% NaCl. These strains also increased peanut productivity under both saline and control conditions[67]. It has been observed that the alkaliphilic bacteria Planococcus rifietoensis increases the growth and production of wheat crops under SS[80,81].
Zahra et al. investigated the genetic diversity of ST-PGPR that was isolated from the wheat rhizosphere[82]. The majority of the isolates, they discovered, belonged to the species Bacillus and could withstand concentrations of up to 8% NaCl. According to Kothari et al., Bacillus safensis VK from Gujarat, India's desert demonstrated a pH range of 4 to 8 and a salt tolerance of up to 14% NaCl[83]. Around 121 bacterial strains were isolated from tsunami-affected areas in the Nicobar and Andaman Islands of India; 23 of these strains exhibited PGP characteristics, such as the production of extracellular enzymes, siderophores, IAA, and PO43− solubilization, and showed salt tolerance up to 10% NaCl[84]. According to the study, Lysinibacillus sp., Alcaligenes faecalis, Microbacterium resistance, Enterobacter sp., and Bacillus sp. accounted for the remaining isolates[84]. Zhang et al. reported on the diversity of ST bacteria that were isolated from the paddy rhizosphere in Taoyuan, China[85]. They discovered that adding salt to the culture medium enhanced auxin levels, indicating that it may be employed as an effective bio-fertilizer in saline circumstances. Yao et al. reported that ST-PGPR strains were effective in balancing the amount of phytohormones generated by plants to improve their stress tolerance[86]. They found that inoculating cotton seedlings with the ST-PGPR strain Pseudomonas putida Rs-198 boosted endogenous IAA production, enhancing the development of cotton seedlings under SS. Aside from the aforementioned Pseudomonas sp., several Bacillus strains can also help plants grow better under stressful situations[2]. In maize seedlings growing in salinized soil, Bacillus amyloliquefaciens SQR9 was shown to improve salt tolerance. Increased chlorophyll, glutathione, and total sugar content, as well as POD and CAT activity were all seen after bacterial inoculation hereby maintaining the K+/Na+ ratio[87]. In comparison to control plants, priming IAA-producing Pseudomonas knackmussii MLR6 to Arabidopsis thaliana boosted plant development and decreased oxidative damage induced by SS[88]. ST-PGPR has shown promise in decreasing plant ACC levels by directly absorbing ACC from plant roots and converting it to α-ketobutyrate and NH3, according to studies[2,45]. This reduces the amount of ethylene in plants, which may otherwise cause serious harm[2,89]. In comparison to groundnut seedlings primed with ACC lacking Pseudomonas strains, Saravanakumar and Samiyappan found that priming groundnut seedlings with ACC-containing PGPR strain Pseudomonas fluorescens TDK1 enhanced plant growth and stress tolerance in salt-affected plants[90]. Similarly, Shahzad et al. found that ACC-containing rhizobacteria increased the number of lateral roots, lateral root length, and dry root weight in salt-stressed crops[91]. It has been discovered that bacterial ACCD aids in the development of a variety of agricultural plants that are subjected to SS[2,92]. Rhizosphere bacteria create osmolytes, which enable plants to adapt to highly salty environments, in addition to phytohormones. Upadhyay et al. investigated the effects of PGPR inoculation on wheat rhizosphere development and found that co-inoculation of Bacillus subtilis SU47 and Arthrobacter sp. SU18 significantly increased wheat plant dry biomass, soluble sugars, and proline content[93]. The inoculation of Bacillus sp. on pea seedlings increased the antioxidant enzymes thereby protecting the plants from oxidative burst and cellular damage under salt stress environment[2,94]. In another study, Khan et al. examine how P. fluorescens and methyl jasmonate work together to strengthen mustard plants (Brassica juncea) resistance to abiotic stress by enhancing their antioxidative defense systems, increasing the production of secondary metabolites, and encouraging the accumulation of osmolytes[95]. Antioxidants, on the other hand, help plants cope with SS[1,2,8,10,12,73,96].
Production of auxins
-
Phytohormones, which include auxins and ACCD, are a class of plant growth regulators that boost plant development even at low concentrations. These microbial compounds have a direct impact on plant development by altering a variety of physiological and metabolic activities in metal-stressed plants. Plant development was increased by the administration of ACCD-positive bacteria, and Enterobacter sp. relieved SS in Cajanus cajan[97]. Auxins are important for the induction and proliferation of the root system of plants. Auxin production is a frequent characteristic of ST-PGPR, and it is thought to be a powerful SS reliever in plants. Auxin regulates various cellular activities in plants, including vascular tissue differentiation, lateral and adventitious root initiation, stem and root elongation, and root and shoot growth direction in response to light and gravity[98]. Rhizobacterial strains produce a variety of auxins, including indole-3-acetic acid (IAA), indole-3-butyric acid (IBA), indole-3-propionic acid (IPA), phenylacetic acid, and others. IAA is the most significant and well-known auxin molecule, which promotes plant development by encouraging root growth through cell division and tissue expansion, vascular bundle production, root nodule formation, and seedling vigor[16,99]. Inoculation of rhizobacterial strains with IAA activity improves root hair development and root growth by enhancing cell elongation and division of diverse agricultural crops, according to Karthik & Arulselvi[100]. ST-PGPR increases plant cell growth and proliferation when combined with the endogenous IAA pool found in plants. Plant root and leaf development rates were shown to be maintained in auxin-producing ST-PGPR strains that survived SS. This is because ST-PGPR produces more auxin, which is then transmitted to the surrounding rhizosphere[101]. Interestingly, phytohormones produced by PGPRs appear to protect against the harmful effects of HMs[102]. Inoculation with IAA-producing PGPR, for example, led to increased plant growth and Zu and Cu accumulation in Brassica oxyrrhina[103]. Plant growth and development are aided by IAA, IBA, and phenylacetic acid, in addition to IAA[104].
Diverse phytohormones, such as auxin, cytokinin, and gibberellic acid, are secreted by diverse PGPR, including Acinetobacter, Arthrobacter, Ochrobactrum, Pseudomonas, Enterobacter, Rhodococcus, Streptomyces, and Serratia[105]. Auxins, majorly IAA also produced by PGPR, have a noticeable impact on overall plant growth promotion, and the main function of IAA is improvement in nutrient uptake, and mineral as well as root system architecture change, which can lead to an overall increase in total root surface and increases in mineral uptake. IAA can increase nodulation, and PGPR significantly improves abiotic stress tolerance[105]. Auxins have an apparent impact on promoting plant growth. Nodulation can be increased by IAA, and abiotic stress tolerance may be significantly improved by PGPR[105]. Additionally, Streptomyces species can increase the bioavailability of nutrients and mitigate abiotic stressors such as SS, DS, and organic and inorganic pollutants in soil[106,107].
It has been shown that the ACCD-producing ST-PGPR affects various biochemical characteristics of plant cells, such as membrane stability, the generation of biocompatible solutes, and the production of photosynthetic pigments during SS and DS[108]. Both Azospirillum lipoferum and A. brasilense, two of the most significant PGPRs can promote plant development by generating components of plant growth such as auxins and other plant hormones[109].
Nitrogen fixation
-
By reducing or blocking the N-assimilating enzymes, such as nitrate reductase (NR), which converts NO3‒ to NO2‒, and nitrite reductase (NiR), which further converts NO2 to NH4+, SS can limit plants' ability to absorb N[110]. Salt-tolerant rhizobia's symbiotic N fixation is crucial for maintaining the balance of plant N requirements during SS. Due to the inert nature of atmospheric N, plants are unable to utilize it directly. Surprisingly, certain rhizosphere bacterial communities use a complicated enzyme system called nitrogenase to convert atmospheric nitrogen to fixed compounds such as ammonia or nitrate continually[111]. For a brief moment, Rhizobium leguminosarum bv trifolii can fix elemental N in the presence of HMs, which enhances the test crop's root and shoot elongation and biomass[112]. In Nickel-contaminated soil, Kamran et al. found that PGPR inoculation increased N-absorption and boosted plant ROS scavenging activity[113]. They also discovered that the PGPR's N-fixing action enhanced glutathione reductase enzyme production, which decreases peroxide radical toxicity. Aside from promoting plant development, N-fixing rhizobacterial inoculation boosts the plant's phytoremediation efficacy[114]. EPS, HCN, NH3, N-fixation, siderophores, and IAA may all be produced by P. plecoglossicida RGK in turmeric plants[115].
Solubilization of phosphates
-
The phosphate transporters (PHTs), which are found in the plasma membrane, facilitate the uptake of P by plants as phosphate (PO43−)[116,117]. Their expression is impacted by SS, which results in dysfunction and decreased PO43− absorption in the root area[118]. According to certain research, PGPR can enhance PO43‒ in plants under SS and modify the expression of a few important genes linked to PO43− transport[119,120]. Genes for phosphatase activity and the synthesis of organic acid are discovered to be overexpressed in ST-PGPR, which aids in the enhanced solubilization of insoluble mineral phosphate. For example, it was discovered that inoculating P. putida MTCC 5279, an ST-PGPB, increased acidic phosphatase activity in phosphate and salinity-starved conditions[121].
Plants were unable to use PO43‒ directly from the soil because it existed in an insoluble state. However, by the secretion of enzymes (phosphonates, phosphatases, and carbon-phosphorus lyases), organic acids (citric acid, lactic acid, and gluconic acid), and certain soil bacteria play an essential role in PO43‒ solubilization[122]. Some metal-resistant endophytes increase PO43‒ mobilizations under P-limiting conditions by secreting extracellular phytase, which improves nutrition availability in HM-stressed rhizospheric regions[102]. According to Motesharezadeh et al. inoculation of PO43‒ solubilization PGPR strains Pseudomonas and Bacillus sp. improves plant development by enhancing soil nutrition quality[102]. Several studies have previously shown that PO43‒ solubilizing PGPR strains can promote plant development when exposed to HMs[123]. According to Rojas-Solis et al. Pseudomonas plecoglossicida RGK was discovered to have the ability to solubilize K, Zn, and PO43‒[115]. After being isolated from tomato plants, P. aeruginosa strain FG106 produced siderophores, NH3, IAA, HCN, and PO43− solubilization[124]. According to Lim et al. Serratia fonticola DSM 4576T can also be involved in the glutamate synthase (GS/GOGAR) pathway, which is responsible for the solubilization of inorganic PO43‒, HCN synthesis, IAA production, siderophore production, and NH3 assimilation[125]. Due to its ability to promote plant development through PO43‒ solubilization and the synthesis of stress-relieving enzymes, Pseudomonas sp. RGM 2987, which was isolated from Stevia philippiana roots, was launched as a novel plant biostimulant[126].
Other plant growth-promoting properties and 1-aminocyclopropane-1carboxylate (ACC)
-
Ethylene is a plant growth regulator that is produced in response to a variety of environmental stresses, including HMs, SS, DS, floods, pathogen attachment, and so on[8]. The enzyme ACC synthase is important in the production of ethylene. Ethylene is involved in plant development and other metabolic functions in small amounts[2,127]. However, when plants are stressed, their ethylene levels rise considerably, and when they reach a high enough concentration, it can disrupt root development and plant metabolisms, resulting in decreased crop performance. As a result, controlling the amount of ethylene in plant tissues is crucial. In this situation, PGPR strains that produce ACCD serve a key role in controlling ethylene levels in plant tissues by degrading ACC enzymes[2,127]. ACCD belongs to a broad family of enzymes that convert ACC to α-ketobutyrate and NH3, which plants may use as carbon and nitrogen sources and are resistant to environmental stress[2]. Furthermore, by reducing ACC, PGPR strains reduce the negative effects of ethylene and stimulate plant development[75]. The inoculation of rhizobacterial strains with ACCD activity improves a variety of plant development metrics as well as phytoremediation effectiveness[128] thereby increasing plant health and growth. These helpful microorganisms lessen the effects of SS, which usually prevents plants from absorbing water, shortens their cell elongation, and stunts their development. By maintaining the equilibrium between Na+ and Cl− and halting the depletion of vital nutrients like Ca2+, K+, H+, N, and NO3−, they mitigate the effects of ionic stress. Furthermore, ST-PGPR (ACCD positive) maintains antioxidant levels by avoiding damage to proteins, lipid membranes, DNA, and RNA as well as by lowering the production of ROS in response to oxidative stress. Because ST-PGPR improves crop yield even in challenging circumstances, its many advantages greatly support sustainable agriculture (Fig. 3).
Figure 3.
Effect of ST-PGPR on plant growth, health, and stress tolerant. Salinity stress (inhibition of water uptake, reduction of cell elongation, and decrease in leaf growth), ionic stress, and nutrient imbalance (increase in Na+ and Cl− levels, reduction in Ca2+, K+, H+, N, and NO3− levels, and accelerate leaf senescence); oxidative stress (generation of ROS, DNA/RNA/protein/lipid membrane damage, depletion of antioxidants). ST-PGPR: salt-tolerant plant growth-promoting rhizobacteria; EPS: Exopolysaccharide; ACC: 1-aminocyclopropane-1-carboxylate; Na+: sodium; Cl−: chloride; K+: potassium; H+: proton.
When the ACCD-producing rhizobacterial strains Klebsiella Mc173, Serratia K120, Escherichia N16, and Enterobacter N9 are employed as bioinoculants, they increase the shoot and root length of Helianthus annuus and thereby increase the phytoremediation of Cu, Ni, Zn, Pb, and As[129]. ACC is synthesized in the plant in response to stresses such as SS, DS, HMs, waterlogging, temperature extremes, pesticides, and others[92]. The acdS gene encoding the ACCD enzyme is essential for controlling plant ethylene levels and reducing stress-related damage. This gene is made up of several regulatory elements that work together to guarantee that its expression is strictly regulated and sensitive to environmental factors. PGPR strains that produce ACCD have been identified and made use of recent developments in molecular biotechnology and omics techniques including metagenomics, transcriptomics, proteomics, and next-generation sequencing. By using these cutting-edge methods, scientists have been able to find PGPR strains that can flourish in harsh environments, greatly increasing plant resilience and tolerance to a range of stresses[92]. The development of sustainable farming methods that can survive the mounting difficulties brought on by environmental stressors such as climate change is greatly encouraged by this success.
-
There must be an intimate link between the PGPR and the host plant for PGPR to have a favorable influence on plant development via an elevation of the nutritional status of their host. However, depending on where and how the PGPR colonizes the host plant, the degree of closeness between the PGPR and the host plant might vary. Rhizospheric is one type of relationship between PGPR and its hosts. PGPR may colonize the rhizosphere, the root surface, or even superficial intercellular gaps in rhizospheric interactions (Table 2), although this latter situation may often involve dead cell layers.
Table 2. PGPRs and their relationships to hosts on various crops.
PGPR Relationship to host Host crop Ref. Azospirillum sp. Rhizospheric Zea mays and O. Sativa [116,130] Azotobacter sp. Rhizospheric Z. mays and Triticum aestivum [128,131] Bacillus subtilis Rhizospheric T. aestivum [132,133] Burkholderia sp. Endophytic O. sativa [134] Cyanobacteria Rhizoshperic O. sativa and T. aestivum [135,136] Gluconacetobacter diazotrophicus Endophytic Sorghum bicolor and Saccharum officinarum [137,138] Bacillus cereus and Bacillus marisflavi Rhizospheric Pisum sativum and Zea mays [16,73] These locations are inaccessible to any soil bacteria. The plant, by definition, alters the physical and chemical composition of the soil in the rhizosphere in comparison to the bulk soil, which might impair a PGPR's capacity to colonies the rhizosphere. Due to plant exudations, changes in soil pH, water potential, partial pressure of O2, and a variety of other physical and chemical features reveal themselves[139]. Gong et al. demonstrated that Streptomyces sp. KLBMP5084 has a high potential for promoting tomato seedling growth as the biological fertilizer under SS; they also reported a significant decrease in malondialdehyde, as well as the increasing of proline contents, soluble sugar, and antioxidant enzyme activity in both the stem and leaf of tomato plants[140].
Antioxidant shielding to mitigate oxidative stress and reduce salinity stress
-
For ST-PGPR, plants have sophisticated identification and communication mechanisms. Many processes are involved in this relationship, including chemical signaling, quorum sensing, root exudates, chemoattraction, biofilm formation, mycorrhizal connections, and plant immune responses[141]. One important way that plants detect ST-PGPR is by chemical communication. Plants release a wide range of chemicals, such as organic acids and flavonoids into the rhizosphere—the region of soil that surrounds the roots of the plant. These compounds attract ST-PGPR to the root zone by acting as signaling molecules[142]. According to Bhat et al., rhizospheric microbes—particularly ST-PGPR—play a critical role in helping plants adapt to SS by maintaining the osmotic balance, ion homeostasis, EPS production, enhancing the plant antioxidant defense system, and nutrient cycling[143].
When hypersalinity stress signaling happens between the rhizospheric microbiome and plants, it is even more crucial to comprehend the processes involved[28,144]. Typically, plant signal transduction begins with the perception of the signal by surface receptors, which then produce ROS and inositol phosphates as secondary messengers. The secondary messengers that work upon Ca-dependent protein kinase (CDPK), microtubule-associated mitogen-activated protein kinase (MAPK), and protein phosphatases regulate Ca2+ ion regulation, which in turn controls gene expressions under SS[18]. This involves a significant number of transcription factors (TFs) from families that affect genes involved in SS signaling, including bZIP, MYB, AP2/ERF, and NAC. Most of these modulations are solely post-transcriptional.
Numerous PGPB and endophytes have shown that under various stress conditions, induction genes of signaling can occur. Two crucial pathways that plants use to combat environmental stress are MAPK and CDPK. Since MAPK (MAPK, MAPKK, and MAPKKK) is extremely conserved, signal transmission occurs via the phosphorelay mechanism. Different signal molecules can activate MAPK, and within these pathways, cross-talk may take place to improve SS tolerance. Under SS, cells often react to their surroundings fast through the presence of Ca-sensing proteins (such as calmodulins and CDPK) and by adjusting the amount of Ca2+ ions in the cytoplasm. Plants produce less ROS and ABA when they are under SS owing to CDPK. ST-PGPRs interfere with several processes by controlling ion homeostasis and absorption, which helps plants become resilient to SS. Therefore, several ST-PGPR genes and proteins (MAPK, RAB18, DREB, and CIPK12) are involved in regulating the defense and hormone production for improved plant adaptation to SS[145]. According to Zhao et al. Arthrobacter sp. ZCY-2 possesses five circular plasmids and a circular chromosome that encodes for the processes of pollutant degradation and salt adaptation, demonstrating its distinct saline tolerance trait[146]. Under SS in Rapeseed, Arthrobacter globiformis can increase phenolic compounds, PAL, and SOD enzymatic activity[147].
According to Ji et al. Enterobacter cloacae HG-1 may enhance wheat's ability to withstand SS and encourage the growth of the plant[148]. The rice host's biochemical and physiological stress responses may be enhanced by Streptomyces albidoflavus OsiLf-2, and it can also significantly boost the host's tolerance to SS[149]. In addition to improving development in non-stressed circumstances, Pseudomonas sp. EB3 may shield banana plants against fusarium and SS[150]. According to Gu et al., P. fluorescens UM270 has demonstrated a functional role in promoting tomato plant development, particularly under SS circumstances where it led to an increase in dry weight, chlorophyll content, and shoot and root length[151]. It is well known that cyanobacteria, or blue-green algae, release a variety of compounds that affect the growth and development of plants. These chemicals include auxin, gibberellin, ABA, cytokinin, vitamins, polypeptides, and amino acids, among other growth-promoting regulators[152].
In an SS environment, plants produce more ROS such as single oxygen (O2), hydroxyl radical (OH·), superoxide anion (O2·−), and hydrogen peroxide (H2O2)[8]. As a result, essential biomolecules including photosynthetic pigments, lipids, and proteins suffer oxidative damage along with the degradation of cell membrane integrity due to polyunsaturated fatty acid oxidation in the lipid layer, which ultimately results in plant death (Table 3).
Table 3. Influence of plant growth-promoting rhizobacteria (PGPR) on gene expression regulation under SS conditions.
PGPR Plant species Expressed gene Role of gene expression under SS Ref. B. megaterium Zea mays ZmPIP Increased root hydraulic conductivity [153] B. amyloliquefaciens O. sativa NADPMe2, EREBP, SOS1, BADH,
and SERK1Increased plant growth and salt tolerance [154] Pseudomonas putida Solanum lycopersicum Toc GTPase Imported chloroplast protein [155] Enterobacter sp. Solanum lycopersicum DREB2b, RD29A, RD29B, RAB18, P5CS1, P5CS2, MPK, and MPK6 Antioxidant activity enhanced; increased
proline biosynthesis[156] B. amyloliquefacis Zea mays RBCS, RBCL, H+-PPase, HKT1,
NHX1, NHX2, and NHX3Promoted seedling growth and Chl content; Improved POD/CAT activity and glutathione content; Decreased Na+ toxicity [87] Dietzia natronolimnaea Triticum aestivum APX, MnSOD, CAT, POD, GPX, GR, TaABARE, TaOPR1, TaMYB, and TaWRKY Increased proline content and antioxidant enzymes [157] Enterobacter sp Abelmoschus esculentus and Arabidopsis sp. APX and CAT Increased antioxidant enzyme activities [158] Arthrobacter protophormiae and B. subtilis Triticum aestivum TaCTR1 and TaDRE2 Enhanced salt stress tolerance [159] Pseudomonas simiae
strain AUGlycine Max L. DREB2A, EREB, GOLS, and
P5CS, PIP, and TIPUp-regulated TF, osmoprotectants, and water transporters by enhancing drought and salt tolerance by regulating stress-responsive genes [160] Bacillus sp. SR-2-1/1 Zea mays RBCL, CAT1, APX1, APX2, NHX1, SOS1, H+-PPase, and HKT1 Enhances photosynthesis efficiency, maintains ion homeostasis, regulates vacuolar proton pump, and minimizes oxidative stresses [161] Bradyrhizobium japonicum
and Enterobacter Delta PSKGlycine Max ‒ Enhanced the morphological parameters and chl a, decreased the electrolyte leakage, malondialdehyde, and H2O2 thereby mitigating OS [162] P. aeruginosa HG28-5 Solanum lycopersicum L. NCED, ABF4, ABI5, and AREB Regulating Na+/K+ homeostasis and ABA signaling pathway [48] ‒ Arabidopsis thaliana SOS1 and HKT1 Regulate efflux of Na+ ad enhancing SS tolerance [163] Beijerinckia fluminensis Arabidopsis thaliana SOS1, NHX1, and MYB genes Modulation of SS tolerance using signal response pathways and regulating ion homeostasis [164] SS: Salinity stress; RBCS: encodingribulose-1,5-bisphosphate carboxylase/oxygenase (Rubisco) small subunit; RBCL: encoding ribulose-1,5-bisphosphate carboxylase/oxygenase largesubunit; H+-PPase: encoding H+-pumping pyrophosphatase; HKT1: encoding high-affinity K+ transporter 1; NHX: encoding Na+/H+ antiporter; ABARE: ABA-signalling cascade; PIP: Plasma membrane intrinsic protein; CTR: Copper uptake protein; DRE2: Dehydration responsive element 2; APX: Ascorbate peroxidase; SOD: Superoxide dismutase; CAT: Catalase; POD: Peroxidase; GPX: Glutathione peroxidase; GR: Glutathione reductase; OPR1: Oxophytodienoate reductase; MYB: Myeloblastosis; Toc GTPase: Translocons of the outer; DREB2b: Dehydration-responsive element-binding protein 2B; RD29A: Response to Desiccation; RAB18: Ras-related protein; P5CS1: Delta1-pyrroline-5-carboxylate synthase 1; MPK: MAP kinase; NADPMe2: NADP-malic enzyme; EREBP: Ethylene-responsive element binding protein; SOS1: Salt-overly sensitive; BADH: Betaine aldehyde dehydrogenase; SERK1: Somatic embryogenesis receptor-like kinase 1; DREB2A: Dehydration-responsive element binding protein2a; EREB: Ethylene responsive protein; GOLS: Galactinol synthase; P5CS: 1-Pyrroline-5-carboxylate synthetase; PIP: Plasma membrane intrinsic protein; TIP: Tonoplast Intrinsic Proteins; RBCL: Ribulose bisphosphate carboxylase large chain; OS: Osmotic stress; NHX1: Sodium/hydrogen exchanger 1; SOS1: Salt Overly Sensitive 1; H+-PPase: Proton-pumping pyrophosphatase; NCED: 9-cis-epoxycarotenoid dioxygenase; ABF4: ABRE (abscisic acid-responsive elements) binding factor 4; ABI5: ABA-insensitive5; AREB: ABA-responsive element binding protein. Rhizo-microbiomes use a strong antioxidant defense system to fight off the harmful effects of ROSs in plants. This defense system includes the secretion of antioxidant enzymes such as APX, CAT, and SOD as well as non-enzymatic low-molecular-weight antioxidant compounds like proline, β-carotenoids, α-tocopherol, ascorbic acid, and glutathione. When Azotobacter sp. was applied rhizospherically to Glycyrrhiza glabra L., an endangered plant with significant medicinal and industrial value, it showed a high expression of antioxidants like APX, CAT, PPO, POD, and PAL at salinity levels (200 mM)[165]. Pisum sativum's SS is lowered by Bacillus and Pseudomonas sp. by promoting the activity of SOD, CAT, POD, PRP, SOD, and others[2,45].
Gene expression profiling in plant responses triggered by ST-PGPR
-
In agriculture, PGPR's ability to support plant growth and provide tolerance to a range of stresses has garnered a lot of interest. Knowing the molecular processes behind the beneficial interactions between plants and PGPR is crucial to maximizing the use of plants in sustainable agriculture. Complex reactions are caused by PGPR in plants, and comprehending these processes requires the use of a powerful molecular technique termed gene expression profiling (GEP)[51]. GEP is a powerful molecular technique that includes the examination of alterations in gene expression patterns following PGPR vaccination and allows researchers to look at the activity of individual genes in a cell or tissue at a given point in time. One way to determine whether genes are up-regulated (switched on) or down-regulated (switched off) is to measure the amounts of RNA molecules, such as messenger RNA (mRNA). By providing a snapshot of gene activity using technologies like microarrays or next-generation sequencing, this approach helps researchers better understand how genes respond to various conditions, diseases, or treatments. GEP is critical in fields such as genomics, personalized medicine, and understanding the molecular principles underlying complex biological processes[24]. This technique allows researchers to identify genes that either up or down-regulate the expression of PGPR through interaction. These genes often have varying expression patterns, but they are important for plant growth, development, and stress tolerance. Analyzing these genetic alterations helps scientists better understand how PGPR benefits plants. GEP sheds light on the signaling pathways involved in the crosstalk between plants and ST-PGPR. It enables the identification of crucial genes and regulatory molecules that mediate the symbiotic relationship[166]. With this knowledge, new strategies for increasing agricultural output and resilience to environmental stresses can be developed. This approach not only advances our knowledge of the interactions between plants and ST-PGPRs, but also opens up new avenues for using these beneficial bacteria in resilient and sustainable agriculture[87].
Regulation of gene expression through transcriptional control and epigenetic modifications
-
The basic process of gene expression in biology regulates the production of proteins that carry out essential functions in cells. To ensure that the required genes are activated or inactivated at the appropriate times and in response to signals, it is tightly controlled. Transcriptional control, or the regulation of gene expression at the transcriptional level, and epigenetic modifications, or heritable changes in gene activity without modifications to the DNA sequence, are two key mechanisms that play a part in this complex dance of genetic information. When combined, they offer an intricate regulatory network that regulates gene expression and is necessary for development, differentiation, and illness[167]. Transcriptional regulation is the process by which a gene's DNA is transformed into RNA and then translated into a functional protein. This process involves several intricate steps and is highly controlled. Transcriptional regulation is primarily governed by TFs, which are proteins that attach to DNA regions called enhancers or promoters. These TFs can either stimulate or repress gene expression by drawing the RNA polymerase enzyme to the target gene's promoter region. Numerous stimuli, including hormones, developmental cues, and environmental signals, can influence how TFs bind to enhancers or promoters. This dynamic interplay between TFs and regulatory elements enables the precise regulation of gene expression in response to changing physiological conditions[168].
On the other hand, epigenetic modifications involve chemical changes to the DNA molecule and its partner proteins, including histones. These changes have a major effect on gene expression but do not affect the underlying DNA sequence. These changes could be heritable and permanent, which implies that they might be passed down from parent cells to offspring during cell division. Among the significant epigenetic modifications are histone methylation, acetylation, and DNA methylation. DNA methylation, which typically involves the addition of a methyl group to a cytosine base in a CpG dinucleotide context, is often the cause of gene suppression. Histone acetylation and methylation can affect gene expression in an activating or repressive way, depending on the specifics[169].
Plants regulate their gene expression by a complicated and dynamic process that is regulated by various processes, such as epigenetic changes and transcriptional control. The interaction of epigenetic factors and RNA m6A modification, which jointly control the exact expression of genes required for plant growth and stress responses, is a crucial component of this regulation. An important part of mRNA stability, splicing, and translation is RNA m6A modification, a common and reversible chemical alteration[170]. To fine-tune gene expression, this alteration frequently interacts with chromatin remodeling, histone changes, and DNA methylation. The control of gene expression depends on the intricate relationship between transcriptional regulation and epigenetic modifications. The interplay among these processes assures the strict regulation of gene expression, allowing plants to adjust to fluctuating environmental circumstances and sustain their growth and development. Researchers can get a better grasp of the regulatory networks supporting plant physiology and resilience by comprehending these complex relationships[170,171].
TFs can change gene activity by attracting and attracting enzymes that add or remove epigenetic marks. For example, TFs can recruit histone acetyltransferases (HATs) to create histone acetylation, which is linked to open chromatin and active transcription. Histone deacetylation, which is brought on by repressive TFs that enlist histone deacetylase (HDACs), inhibits gene activity. DNA methyltransferases (DNMTs) can be drawn to genes, much like TFs can, which results in DNA methylation and gene suppression. Because of the intricate relationships between transcriptional control and epigenetic modifications, cells can precisely adjust their patterns of gene expression in response to a wide range of signals and stimuli[172]. Epigenetic modifications occur throughout critical stages of development and differentiation. They are essential for the establishment of cell identity because they imprint patterns of gene expression. For example, throughout embryonic development, DNA methylation patterns are regularly changed and subsequently restored in a cell type-specific manner to ensure optimal tissue differentiation. Histone modifications facilitate the emergence of specific cell types by regulating which genes are accessible. Dysregulation of these epigenetic mechanisms can also lead to developmental defects[168]. It is essential for cellular reactivity to stimuli and adaptation. Stress-responsive TFs can trigger the DNA methylation patterns and histone modifications of genes associated with the physiological stress response. These changes help cells adapt to external stresses by changing the expression of genes involved in stress defense and survival[173].
-
A revolutionary path to improving ST-PGPR strains through increased effectiveness and the creation of novel variations is provided by genetic modification. The incorporation of genetic innovations has the potential to improve plant stress response through PGPR-biotechnologies, promoting sustainability, efficacy, and environmental friendliness. Japan's emphasis on intelligent nanomaterials makes it possible to provide agricultural inputs precisely and effectively, reacting quickly to environmental cues and guaranteeing ideal plant development. This advanced utilization of nanomaterials in conjunction with ST-PGPR has great potential[174]. According to Shalaby et al., this interaction is essential for disease detection, nutrient absorption in agriculture, and building organismal resistance to biological and environmental stressors [175,176]. Genetic manipulation, often known as genetic engineering, is the intentional modification of an organism's genetic material, typically DNA. The beneficial traits of strains in ST-PGPRs are also enhanced by genetic engineering[177]. PGPR strains genetic makeup may be changed by applying strategies like CRISPR-Cas9, which allows for precise alterations to be made to bacterial DNA. The synergistic benefits of nanotechnology and ST-PGPR may raise the overall efficiency of agriculture through the strategic integration of nanomaterials. Chemicals produced by PGPR may be precisely distributed to plant roots through the use of nanoparticles as delivery vehicles[178]. By managing the release of nutrients, we can optimize absorption when we combine PGPR-enhanced nutritional availability with nanomaterials like nano-fertilizers.
-
In order to fulfill the world's food demands while reducing the negative social and environmental effects of conventional agricultural methods, sustainable agriculture is crucial. Enhancing agricultural sustainability may be achieved through the practical application of ST-PGPR. Plant growth and health are supported by ST-PGPR, which are naturally occurring soil bacteria that work in symbiotic relationships with plants to help with nutrient absorption, disease resistance, and stress tolerance. Enhancing plant nutrient absorption and availability is one of ST-PGPR's main contributions to sustainable agriculture[179]. These helpful bacteria can solubilize PO43‒, fix N from the atmosphere, and boost the availability of essential nutrients like iron and potassium. As a result, ST-PGPR lessens the need for chemical fertilizers, which are a major cause of greenhouse gas emissions, water pollution, and soil erosion. This reduces the environmental effect of agriculture and lowers production costs for farmers, which is especially helpful in places with limited resources. Other important goals of ST-PGPR includes decreasing the usage of hazardous pesticides and increasing plant disease resistance. When plants are exposed to different strains of the ST-PGPR, they create antimicrobial compounds and gain systemic resistance, which lessens their vulnerability to harmful illnesses. As a result, fewer chemical pesticides are required, which might be harmful to human health, non-target creatures, and ecosystems.
In addition to enhancing soil health and lowering erosion, ST-PGPR also contributes to soil structure and nutrient cycling[180]. They produce organic acids and enzymes that encourage the decomposition of organic matter, increasing the availability of nutrients for plants. They also improve soil aggregation, water retention, and aeration. Higher agricultural productivity and less land degradation are a result of these gains in soil quality, and they are crucial elements of sustainable agriculture[17]. Crops protected by PGPR are more resilient to stressors brought on by climate change, including DS, SS, HMs, and HT[10,73]. This is especially crucial in light of climate change since erratic weather patterns can lead to crop failures and unstable food supplies. By reducing the effects of stresses connected to climate change and maintaining consistent crop yields, ST-PGPR promotes food security and agricultural sustainability. A harmonious relationship between agriculture and the environment is fostered by PGPR-based methods, which emphasize ecological balance, biodiversity, and low chemical inputs. These techniques are in line with the tenets of agroecology and organic farming.
Nevertheless, to fully realize ST-PGPR's promise of sustainable agriculture, various important obstacles need to be overcome. To determine and describe ST-PGPR strains appropriate for various crops, soil types, and climates, more investigation is required. It is possible to increase the effectiveness of ST-PGPR inoculants by tailoring them to particular farming conditions. For field findings to be dependable and consistent, application methods and doses must be optimized[181]. There are inherent limits to the existing study. Finding consistent results is difficult since different experimental conditions exist. The heterogeneity of plant species, PGPR strains, and environmental factors confound the generalizability of findings[145]. It is difficult to pinpoint general processes underlying PGPR-induced signaling since microbial populations and plant responses to stress are dynamic. Furthermore, our comprehension of PGPR's long-term effects on plant development and stress reduction in actual agricultural situations is limited by the absence of long-term research and field-scale assessments[182].
-
To deal with SS, ST-PGPR has developed several strategies. They can tolerate extreme SS by a variety of processes, including efflux systems, the synthesis and storage of suitable solutes for balancing external osmotic pressure, the production of ROS, secondary metabolites, and other mechanisms. Under SS, some metabolites and genes are involved in preserving cell integrity and plant-microbe interactions. There is still a lot to learn at the biochemical and molecular level about how the ST-PGPR supports themselves and their symbiotic partner during SS, which has several effects on the cells (of both bacteria and plants). ST-PGPR research also shows that they have a lot of promise for improving the productivity of agroecosystems that are suffering from salt concerns. In-depth research targeting gene level expression and functional properties of ST-PGPR engaged in plant growth promotion under SS must be done shortly to build tailor-made bio-formulations for saline soil systems, which are on the rise globally. More precise monitoring and usage of ST-PGPR will be achievable with new tools and technologies like improved remote sensing and Metagenomics that will enable more accurate ST-PGPR monitoring and application.
Future investigations ought to concentrate on modifying ST-PGPR plant interactions by genetic editing and exploring the role of the microbiome in PGPR interactions. Agronomy, genetics, and microbiology should be used in interdisciplinary collaborations as part of ambiguity-resolution procedures. Green biotechnology will have a wide range of positive effects on agro-ecosystems and the rural environment. Understanding the processes of osmo-adaptation in ST-PGPR might be a step toward the long-term objective of raising crop yield in saline agroecosystems. Gaining knowledge about the regulatory networks of ST-PGPR and how they induce SS tolerance in plants might be a useful strategy to reduce SS and increase food production worldwide. Improving the productivity of saline soils will lead to improved soil organic matter content and quality in these agrosystems with low nutritional value, as well as aid in the achievement of food security. By increasing our knowledge, using precision agriculture, and embracing biotechnology developments, we can build more resilient, efficient, and ecologically sustainable agricultural systems that will provide food security while reducing their negative effects on the environment. This can aid in the fight against climate change and in lowering carbon emissions.
-
The authors confirm contribution to the paper as follows: study conceptualization, visualization, project administration & supervision: Gupta A; writing-original draft preparation: Gupta A, Singh R; writing-review & editing: Gupta A (extensive contribution), Singh R, Vandana P, Singh K, Sharma D; resources: Gupta A, Singh R, Sharma D; tables and figure design: Vandana P; formal analysis: Vandana P, Singh K; references collection: Singh R, Sharma D. All authors reviewed the results and approved the final version of the manuscript.
-
The datasets generated during and/or analyzed during the current study are available from the corresponding author on reasonable request.
-
The authors declare that they have no conflict of interest.
-
# Authors contributed equally: Anmol Gupta, Priyam Vandana
- Copyright: © 2024 by the author(s). Published by Maximum Academic Press, Fayetteville, GA. This article is an open access article distributed under Creative Commons Attribution License (CC BY 4.0), visit https://creativecommons.org/licenses/by/4.0/.
-
About this article
Cite this article
Gupta A, Singh R, Vandana P, Singh K, Sharma D. 2024. Enhancing crop productivity with salt-tolerant PGPR: A step towards sustainable farming. Vegetable Research 4: e033 doi: 10.48130/vegres-0024-0032
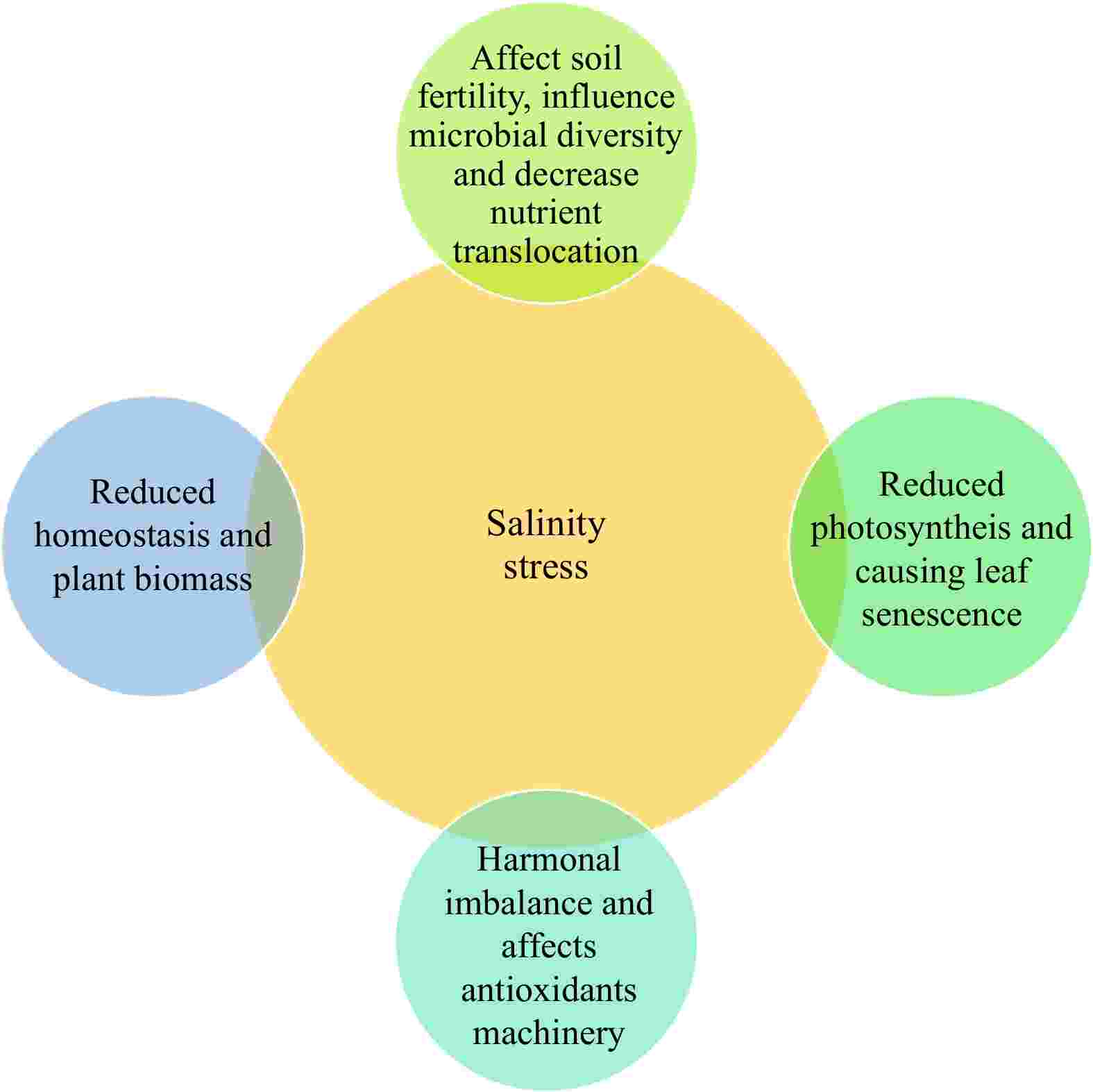