-
The continuous interaction of cadmium (Cd) in the soil and food crop system poses a threat to agricultural production and increases risk of contamination in the food chain[1,2]. Cd toxicity in plants is displayed by inhibition of plant growth, reduced chlorophyll synthesis and carbon fixation, generation of oxidative activity, and peroxidation of membrane lipids[3,4]. A high concentration of Cd in soil generates osmotic activity in plants by disturbing the equilibrium between several physiological parameters[5]. The complementary production of reactive oxygen species (ROS) brings about irreversible damage to biomolecules[6,7]. Additionally, Cd interferes with the uptake of several elements such as calcium (Ca), phosphorus (P), magnesium (Mg), potassium (K), and Manganese (Mn)[8]. A high concentration of Cd in agricultural soil and high mobility of Cd intensifies its toxicity potential and facilitates easy movement from one trophic level to another[9,2].
Zinc (Zn) is an essential micronutrient for plants whose deficiency results in stunted growth, delayed maturity, and reduced yield[10]. Zn plays a role in structural integrity, bio physicochemical responses such as assisting in the metabolism of carbohydrates, lipids, and proteins[11,12]. At high concentrations, toxic effects of Zn have been reported in several plants[13]. Cd and Zn are chemically similar and often compete in root uptake, xylem transport, phloem translocation, and accumulation in edible parts[14,15]. Studies have shown that Zn application decreased uptake, translocation and accumulation of Cd in edible parts[14,16,17]. Zn homeostasis is closely related to that of Cd[18]. A study indicated that Zn had no significant effect on Cd uptake and accumulation[19]. Although Zn is an essential micronutrient, its toxic effects are observed at high concentrations[13,20,21].
The objective of the study was to assess the effectiveness of Zn in mitigating Cd toxicity in spinach (Beta vulgaris L. var. All green). A preliminary experiment was done to assess EC50 Cd dose for spinach (27.42 mg/L)[22], and then the calculated EC50 dose was combined with a series of Zn doses. The response of EC50 Cd-Zn doses was studied to determine if the structural homology of Zn is useful in the management of Cd toxicity in plants; which Zn dose acts as a transitory stage and defines the differentiation between antagonistic and synergistic responses of the Cd-Zn interactions, and what is the mechanistic approach used by spinach for the management of Cd toxicity through Zn application. Antagonistic, or synergistic, impacts of Cd–Zn combination was evaluated through responses of thiol, proline, APX (ascorbate peroxidase), LPO (lipid peroxidation), and ascorbate production.
-
The open-air field experiment was conducted at the Botanical Garden (November 2021−January 2022) of Banaras Hindu University, Varanasi, Uttar Pradesh (25°18' N, 82°1' E; 76.19 m above sea level) in the eastern Gangetic planes of India. The site is characterized by sandy clay loam (45:28:27: sand : silt : clay), pH between 7.20−7.60 ± 0.03, organic carbon content of 0.68%, available nitrogen of 93.04 mg/kg, phosphorous of 12.71 mg/kg, available potassium of 49.67 mg/kg, and Cd of 0.09 mg/kg. The meteorological data such as average maximum temperature (21.4 °C), minimum temperature (9.1 °C), relative humidity (75.5%), and rainfall (16.5 mm) in the study site, collected from the department of Geology, Banaras Hindu University, Varanasi (India). The experiment was arranged in three replicate pots (upper dimension: 28.5 cm, lower dimension: 18.2 cm, and height: 20.3 cm). Each pot was filled with 4 kg of alluvial soil prepared as per local agriculture practices and 10 tons per acre farmyard was added in the pots. Seeds of spinach were procured from the Indian Institute of Vegetable Research (ICAR-IIVR), Varanasi. Six to seven seeds were sown at 2 cm depth in each pot. After emergence, plants were thinned to five seedlings per pot. Water (100 mL per pot once a week) was maintained and regular manual weeding depending upon weed intensity. At 20 d after emergence (DAE), plants were treated with doses of Cd and Zn in the form of CdCl2 and ZnSO4, respectively. The treatments were: (1) CdEC50 (2) CdEC50 + Zn100 (3) CdEC50 + Zn200 (4) CdEC50 + Zn300 (5) CdEC50 + Zn400 (6) CdEC50 + Zn500, and no treatment (control). Where, CdEC50 represents the EC50 Cd dose and Zn100, Zn200, Zn300, Zn400, and Zn500 depict Zn doses of 100, 200, 300, 400, and 500 mg/L, respectively. Each treatment regime was replicated three times. The CdEC50 treatment served as a control for studying the ameliorative effect of Zn in plants grown under treatments 2 to 6.
Plant sampling and analysis
-
For the estimation of biochemical and physiological parameters, triplicate samples per pot were randomly selected at 45 DAE.
Pigment contents
-
Chlorophyll and carotenoid contents were estimated using the formulae of MacLachlan & Zalik[23] and Duxbury & Yentsch[24]. Pigment content was determined by homogenizing 0.5 g of fresh leaves in 20 mL of 80% acetone and then centrifuged at 4,032 × g for 15 min. Optical densities were determined at 645 and 663 nm wavelength for chlorophyll contents, and at 480 and 510 nm for carotenoid contents using a double-beam spectrophotometer (Model-2203, Systronics, Lucknow, India).
ROS production and LPO
-
At 45 DAE, fresh leaf tissue was used for the determination of LPO in terms of malondialdehyde (MDA) content by thiobarbituric acid (TBA) as described by Heath & Packer[25]. For estimation of superoxide (O2·−), At 45 DAE, 0.2 g leaf was extracted in an ice bath in 1 mL 50 mM potassium phosphate buffer containing diethyl di-thiocarbamate (pH 7.8) and then centrifugated at 22,000 × g for 15 min and assay mixture optical density taken at 530 nm[26], with a few modifications. Production of hydrogen peroxide (H2O2) levels was measured according to Alexieva et al.[27].
Enzymatic and non-enzymatic extraction and assays
-
Enzymatic antioxidants superoxide dismutase (SOD), APX, and catalase (CAT) were estimated with procedures described by Fridovich[28], Nakano & Asada[29] and Aebi[30], respectively. Non-enzymatic antioxidants ascorbic acid (AsA), were estimated by following the method of Keller & Schwager[31].
Metabolite contents
-
Total phenolic, thiol, and proline content, were estimated following Bray & Thorpe[32], Arvind & Prasad[33], and Bates et al.[34], respectively. For estimation of total phenolics, a leaf sample was extracted in acetone, using the Folin-Ciocalteu reagent and Na2CO3. Thiol content was determined using Ellman's reagent (DTNB)-5,5'-dithio-bis-(2-nitrobenzoic acid) and 0.02 M EDTA added to fresh leaf extract. For determination of proline content, 0.5 g of fresh leaf tissue was homogenized in 3% sulphosalicylic acid and the mix was filtered through Whatman filter paper (2). Chromophore containing toluene was extracted from the aqueous phase, and absorbance was determined at 520 nm.
Protein content
-
Protein content was quantified using the method of Lowry et al.[35]. Fresh leaf tissue (0.5 g) was extracted in 5 mL tris buffer (0.1 M, pH 6.8) and centrifuged at 5,000 × g for 5 min. Five mL of TCA was added to the supernatant and re-centrifuged at 6,000 × g for 10 min and absorbance was taken at 650 nm on a double beam spectrophotometer (Model-2203, Systronics, Lucknow, India).
Physiological parameters
-
Rates of photosynthesis (Ps) and stomatal conductance (gs) were measured using a Portable Photosynthetic System (Model LI6400XT, Version 6.2, Lincoln, NE, USA). A flag leaf from three randomly selected plants per treatment were chosen for Ps and gs observation. Measurements were done on cloud-free days between 900 and 1,000 h at 45 DAE. Photosynthetically active radiation (PAR) was set at 1,200 μmol/m2/s using a known CO2 source (510 mol/mol concentration) for calibration. Gas measurements were made at a constant flow rate of 500 mmol/s.
Yield
-
Five plants per treatment were selected and the yield was quantified as the fresh weight of the above-ground edible portion of plants.
Statistical analysis
-
All data were subjected to principal component analysis (PCA) and one-way ANOVA using IBM SPSS/PC+ (ver. 25.0, x64, TEAM EQX, MICROSOFT corporation) software. Before performing one-way ANOVA, normality of data was tested by the Shapiro-Wilk test and differences between treatments were above 0.05. The relationships between variables were evaluated using linear regression analysis. Correlation coefficients and bar and staked graphs were drawn between parameters and Cd and combined Cd, Zn dose using Origin 9.0 (Origin Lab, Northampton, MA, USA).
-
Evaluation of toxic effects of CdEC50 on plants, in which the response of plants treated with CdEC50 dose was studied, as compared to the control (no treatment). While studying the effect of Zn, CdEC50 dose-treated plants served as control and CdEC50 + Zn100, CdEC50 + Zn200, CdEC50 + Zn300, CdEC50 + Zn400, CdEC50 + Zn500.
Response of plants treated with CdEC50 dose
-
In plants treated with CdEC50, the increment was observed in SOD (2.43%), APX (4.31%), CAT (11.32%), AsA (10.72%), and LPO (30.46%) and reduction was observed in chlorophyll a (20.43%), chlorophyll b (25.16%), protein (15.41%), and Ps (28.13%) (Fig. 1).
Figure 1.
Assessment of toxic effects of EC50 Cd dose on selected characteristics of Beta vulgaris L. Upper quadrant is showing a positive percentage change and lower quadrant is showing a negative percentage change in enzymatic, nonenzymatic, pigment contents, and physiological parameters during treatments. LPO: lipid peroxidation, H2O2: hydrogen peroxides, O2·−: superoxide, SOD: superoxide dismutase, CAT: catalase, AsA: ascorbic acid, APX: ascorbate peroxidase, Ps: net photosynthetic rate, gs: stomatal conductance, chl a: chlorophyll a, chl b: chlorophyll b, caro: carotienoid.
Response of plants treated with CdEC50 and different Zn doses
Leaf pigment content
-
In plants treated with CdEC50 and Zn doses leaf pigments chlorophyll a, b, total chlorophyll, and carotenoid varied (Fig. 2). Chlorophyll a and chlorophyll b increased by 18%, 42%, 48%, 14%, and 4% and 25%, 42.86%, 46.43%, 32.14%, and 7.14% under CdEC50 + Zn100, CdEC50 + Zn200, CdEC50 + Zn300, CdEC50 + Zn400, and CdEC50 + Zn500 mg/L, respectively, compared to the control (CdEC50). A similar pattern occurred for carotenoid which increased 12.12%, 21.21%, 39.39%, and 3.03% under CdEC50 + Zn100, CdEC50 + Zn200, CdEC50 + Zn300, and CdEC50 + Zn400 mg/L whereas reduced by 9.09% at CdEC50 + Zn500 mg/L, respectively compared to the control (CdEC50) (Fig. 2).
Figure 2.
Variations in pigment contents (chlorophyll a, chlorophyll b, total chlorophyll, and carotenoids) of Beta vulgaris L. plants treated with combined EC50 Cd and doses of Zn at 45 DAE. Bars represent Mean ± S.E. Different colors represent photosynthetic pigments (green; chlorophyll a, yellow; chlorophyll b, pink; total chlorophyll and teal blue; carotenoid) (p < 0.05).
ROS production and LPO
-
There was a decrease in ROS production and LPO. The H2O2 decreased by 7.51%, 10.79%, and 49.76% at CdEC50 + Zn100, CdEC50 + Zn200, and CdEC50 + Zn300 mg/L, respectively, there were increases at CdEC50 + Zn400 (5.16%) and CdEC50 + Zn500 mg/L (14.55%) over the control (CdEC50). The O2·− decreased by 14.71%, 29.41%, 32.35%, and 8.82% at CdEC50 + Zn100, CdEC50 + Zn200, CdEC50 + Zn300, and CdEC50 + Zn400 mg/L, respectively. There was an increase at CdEC50 + Zn500 mg/L by 1.47%. The LPO decreased by 23.23%, 26.38%, and 29.92% at CdEC50 + Zn100, CdEC50 + Zn200, and CdEC50 + Zn300 mg/L. There was an increase at CdEC50 + Zn400 mg/L (4.33%), and CdEC50 + Zn500 mg/L (31.89%) compared with the control (CdEC50) (Fig. 3a).
Figure 3.
Bar graph showing variations in the response of (a) ROS (H2O2; μmol/g FW and O2·−; nmol/min/g), malondialdehyde (MDA) content; nmol/mL. (b) Antioxidant (ascorbate peroxidase; APX; nmol/min/g FW catalase; CAT; μM H2O2 oxidized min−1 g−1 FW, superoxide dismutase; SOD; g−1 FW) and ascorbic acid; AsA; mg/g FW). (c) Protein; mg/g DW, and metabolites (thiol; μM DW, proline; mg/g FW and phenol; mg/g FW) of Beta vulgaris L. plants treated with combined EC50 Cd and doses of Zn at 45 DAE. X-axis shows doses of Zn with EC50 Cd dose. Bar are mean ± S.E. Bars with different letters indicate significant variation at p < 0.05.
Antioxidative response
-
Enzymatic antioxidants increased up to CdEC50 + Zn300 mg/L of APX (3.28%, 7.38%, and 16.40%) and CAT (2.28%, 18.24%, 59.61%); for SOD there was an increase up to CdEC50 + Zn400 mg/L (2.28%, 18.24%, 59.61%, and 6.84%). There were lessened activities of APX (2.46%, 16.39%), CAT (0.66%, 2.5%) and SOD (12.70%, 14.90%) at CdEC50 + Zn400, and CdEC50+Zn500 mg/L, respectively, over the control (CdEC50). Maximum increases occurred in all enzymatic antioxidant pools (APX, CAT, and SOD) at CdEC50 + Zn300 mg/L. The AsA contents decreased in all treatments by 2.33%, 44.19%, 30.23%, and 36.05% at CdEC50 + Zn100 CdEC50 + Zn200, CdEC50 + Zn400, and CdEC50 + Zn500 mg/L, respectively, compared with control (CdEC50) plants. The highest decrease was for CdEC50 + Zn300 mg/L treatments (50%) (Fig. 3b).
Metabolite contents
-
Total phenolic contents declined by 13.19%, 15.35%, and 17.32% at CdEC50 + Zn100 CdEC50 + Zn200, and CdEC50 + Zn300 mg/L. They increased by 10.03% and 33.86% at CdEC50 + Zn400 and CdEC50 + Zn500 mg/L, respectively, compared with control (CdEC50) plants. Thiol content declined by 8.55%, 12.82%, 16.24%, 24.79%, and 74.36% at CdEC50 + Zn100, CdEC50 + Zn200, and CdEC50 + Zn300, CdEC50 + Zn400, and CdEC50 + Zn500 mg/L, respectively, compared with the control (CdEC50). Proline increased by 0.77%, 24.58%, and 32.14% at CdEC50 + Zn100, CdEC50 + Zn400, and CdEC50 + Zn500 mg/L and declined by 0.13% and 0.38% at CdEC50 + Zn200, CdEC50 + Zn300 mg/L, respectively, compared with the control (CdEC50) (Fig. 3c).
Protein content
-
Protein content increased by 17.63%, 35.81%, 39.75%, and 7.66% at CdEC50 + Zn100, CdEC50 + Zn200, CdEC50 + Zn300, and CdEC50 + Zn400 mg/L, but decreased by 14.85% at CdEC50 + Zn500 mg/L, respectively, compared to the control (CdEC50) (Fig. 3c).
Rate of photosynthesis and stomatal conductance
-
The photosynthesis and stomatal conductance (Ps and gs) increased, with greater increases at CdEC50 + Zn300 mg/L. Ps showed by 3.32%, 9.90%, 22.64%, and 1.93% at CdEC50 + Zn100, CdEC50 + Zn200, CdEC50 + Zn300, and CdEC50 + Zn400, but decreased at CdEC50 + Zn500 mg/L (4.47%) treatment. Values of gs increased by 5.71%, 7.86%, and 10.71% at CdEC50 + Zn100, CdEC50 + Zn200, and CdEC50 + Zn300, but decreased at CdEC50 + Zn400 (8.57%) and CdEC50 + Zn500 mg/L (14.29%), respectively, compared with the control (CdEC50) (Table 1).
Table 1. Variation in rate of photosynthesis (Ps; mmol CO2/m2/s) and stomatal conductance (gs; mmol CO2/m2/s) of Beta vulgaris L. plants treated with combined EC50 Cd and doses of Zn at 45 DAE.
Treatment Rate of photosynthesis
(Ps; mmol CO2/m2/s)Stomatal gas conductance
(gs; mmol CO2/m2/s)EC50 16.56cd ± 0.376 1.40c ± 0.25 EC50 + Zn100 17.11c ± 0.664 1.48b ± 0.05 EC50 + Zn200 18.20b ± 0.459 1.51b ± 0.25 EC50 + Zn300 20.31a ± 0.344 1.55a ± 0.09 EC50 + Zn400 16.88cd ± 0.485 1.28d ± 0.11 EC50 + Zn500 15.82e ± 0.437 1.20d ± 0.05 Values are mean ± S.E. Different letters indicate significant variation at p < 0.05. Yield
-
Plant yield increased by 4.01%, 23.06%, 26.57%, and 3.25% at CdEC50 + Zn100, CdEC50 + Zn200, CdEC50 + Zn300, and CdEC50 + Zn400 mg/L, respectively, but decreased by 12.03% at the higher dose of Zn (CdEC50 + Zn500 mg/L), compared to the control (CdEC50) (Fig. 4).
-
Absorption of Cd from the soil by plants expedites toxic effects on plants but results in its inclusion in the food chain. The persistent and bio-accumulative nature of Cd further augments the problem of Cd toxicity in biological systems[36]. Cd toxicity in plants has become a topic of investigation because of its continuously increasing accumulation in agricultural soils owing to the extensive use of fertilizers and pesticides[37,38]. The major cause of Cd toxicity is increased oxidative activity[39], which affects plants at morphological, physiological, biochemical, and molecular levels[40,38]. When applied above a particular dose Cd can produce irrepealable damage to plants[41]. Before establishing strategies aimed at bringing about mitigation of Cd toxicity, it is important to estimate the Cd dose preceding which the repair of plant metabolic functioning can be achieved. Under similar experimental circumstances using biomass measurements EC50 (effective concentration of Cd causing 50% inhibition of plant growth compared to control) was calculated to be 26 mg/L[22]. The inhibitory effect of Cd toxicity on biomass is largely the manifestation of alteration in plant physiological and metabolic activities[42]. The results of the present study indicate that spinach treated with CdEC50 experienced oxidative activity and showed a negative response compared to plants with no treatment. The structural homology between Cd and Zn has been utilized in promoting Zn as a tool for ameliorating Cd toxicity, which, to the best of our knowledge, is the first effort of its kind. Oxidative activity generated by Cd is through enhanced production of important reactive oxygen species like O2·− and H2O2 in CdEC50 treated plants. Studies have shown that Cd-induced oxidative activity is attributed to inhibition of cellular metabolic reactions[43]. Reduction in the ROS content by Zn indicates abatement of Cd toxicity, which is a result of restoration of disruptive metabolic activities under Cd. Since Zn has no direct role in regulating ROS production[44], the reduction of ROS contents in Cd + Zn treated plants is attributed to the abatement of Cd-induced oxidative activity. The MDA levels, which are a potential biomarker of oxidative activity indicated a significant reduction in the CdEC50 + Zn treated plants compared to CdEC50 treated plants, which strengthens the curative property of Zn toward Cd. Reduced MDA contents in plants supplemented with Zn indicates stability to the membrane lipid and protein which supports increased photosynthesis and higher protein content in CdEC50 + Zn treated plants. Higher Zn concentrations (above 300 mg /L) increased MDA in plants indicating the synergistic effect of Zn and Cd at a higher Zn dose. At high Zn concentrations, peroxidation of the lipid component of membranes occurs which results in activation of membrane-localized NADPH oxidase, generating more ROS[45]. The fluctuation in MDA content of Cd and Cd-Zn treated plants can be negatively correlated to their respective ROS contents (H2O2 and O2·−). Whereas CdEC50-treated spinach plants had a higher concentration of H2O2 (28.81%) and O2·− (17.61%), compared to untreated plants; ROS declined substantially in CdEC50 + Zn treated plants, compared to CdEC50 plants. A similar correlation between ROS generation and membrane integrity was also established in Vicia faba L. cv Nubaria at different Cd and Zn concentrations[45].
Zn supplementation reduced Cd damage by stimulating the cellular defense machinery, the effect being most noticeable at Zn 300 mg/L. The SOD, considered to be the first line of defense against abiotic challenge[46] increased the most among all antioxidant enzymes in Zn-supplemented Cd-treated plants. A high level of oxidative exposure tends to inhibit SOD activity[47]. The present result of Cd-treated plants complies with other studies, wherein a non-significant effect on SOD was recorded along with a steep increase in O2·− concentration. Zn supplementation triggered the inactive SOD of Cd-stressed plants, which was evident through the significant increments in the SOD activity, which was as high as 59.60% in CdEC50 + Zn 300 mg/L dose as compared to the CdEC50 dose. Zn is an essential metal prosthetic group up-regulated the Cu-Zn SOD expression, enhancing SOD activity in Cd-Zn treated plants, compared to Cd-treated plants[48].
In the present study, the positive effect of Zn on SOD activity can further be verified by a significant reduction in concentrations of O2·− in CdEC50 + Zn treated plants, compared to CdEC50 treated plants, which experienced a substantial accumulation of O2·−. Activation of SOD due to Zn treatment stopped dismutation of O2·−, thereby reducing its contents in CdEC50 + Zn plants, compared to Cd-treated plants (Fig. 5). The ROS behavior reduced O2·− content was of higher magnitude than that of H2O2 content in Cd-Zn treated plants (Fig. 5). This response of ROS can be due to the disparate stimulation of enzymatic antioxidants with Cd-Zn treatment. In the present study Zn supplementation, although an increment in the activities of all the studied enzymatic antioxidants was recorded, the enhancement was less in H2O2 scavenging enzymes like APX and CAT as compared to SOD (0.82), (Fig. 5), which are responsible for the dismutation of O2·− to H2O2. Lesser reduction of H2O2 to O2·− in CdEC50 + Zn treated plants, compared to CdEC50 treated plants, can be accounted for through less efficiency of APX and CAT and higher feedback of SOD upon Zn treatment. An extrapolation of responses of CAT and APX in the present study indicated CAT (0.81) to be a more efficient scavenger of H2O2 in Cd + Zn treated plants. This is further evident through correlation values (R) and regression value (R2), which was higher for CAT (R = 0.97, R2= 0.94), compared to APX (R = 0.90, R2 = 0.82) (Fig. 6), and in PCA evident, higher synchronization of CAT (0.81) and SOD (0.82) (Fig. 7). This observation contradicts earlier literature as APX has a higher affinity toward H2O2 compared to CAT[49]. In the present study, Zn treatment was not able to revive the APX activity of Cd-treated plants, it is also unable to sustain AsA regeneration, which is crucial in scavenging H2O2. The antagonistic effect of Zn treatment towards antioxidant response in CdEC50 + Zn treated plants was only up to 300 mg/L Zn, after which Zn and Cd act synergistically leading to enhanced negative effects on plants. Significant reductions in thiol contents in CdEC50 + Zn treated plants indicate a decline in the GSH pool which can be correlated with a disturbed continuity of the AsA-GSH cycle. This explains the reduction in AsA in CdEC50 + Zn treated plants compared to CdEC50 treated plants. The present results suggest that the AsA-GSH cycle may not play a significant role in imparting Cd tolerance to spinach plants upon Zn supplementation, due to inefficiency of sustenance of the GSH pool. Antioxidants response of Cd EC50 + Zn treated spinach plants suggests the role of SOD and CAT activity is more decisive in providing tolerance towards Cd, upon 300 mg/L dose of Zn. Higher Zn concentration acted synergistically with Cd, denigrating the positive response of antioxidative enzymes. Stomatal regulation is an important biophysical aspect of plants that determines their sensitivity toward abiotic challenges[42]. In the present study, although Cd application reduced stomatal conductance, Zn application in CdEC50 + Zn plans favor stomatal opening. Zn promotes stomatal regulation owing to its positive effect on membrane permeability which sustains the K+ transportation across the guard cell membrane, a feature that was disrupted due to Cd[50]. In addition, Zn treatment favors the accumulation of osmolytes in Cd-treated plants which assist in stomatal opening[50]. The positive effect of Zn extends to carbon fixation, as evident by increased rate of photosynthesis in Cd-treated plants.
Figure 5.
Comparative analysis of the effect of treatments (Control-CdEC50 and CdEC50-CdEC50 combined with doses of Zn) on ROS (H2O2 and O2·−) generation, lipid peroxidation (LPO), antioxidative response, rate of photosynthesis, secondary metabolite and protein contents of Beta vulgaris L. plants. The color gradient of the heatmap shows the variation in ROS, antioxidants and secondary metabolites. Lighter colors are showing lower concentration and dark colors are showing higher concentration. The pathway is showing conversion of antioxidant machinery with the color gradient of the heatmap showing lower (light color) to higher concentrations (dark color) of antioxidants. Legend numbers represent the concentration of antioxidant machinery. LPO: lipid peroxidation, H2O2: hydrogen peroxides, O2·−: superoxide, SOD: superoxide dismutase, CAT: catalase, AsA: ascorbic acid, DHA: dehydroascorbate, MDHA: monodehydroascorbate, GSH: glutathione, GSSG: glutathione, APX: ascorbate peroxidase, DHAR: dehydroascorbate reductase, MDHAR: monodehydroascorbate reductase, GR: glutathione reductase, NADP reductase; NADP: nicotinamide adenine dinucleotide phosphate; NADPH: reduced nicotinamide adenine dinucleotide phosphate, ROS: reactive oxygen species, Ps: net photosynthetic rate, gs: stomatal conductance.
Figure 6.
Regression analysis to estimate the correlation of H2O2 with ascorbate peroxidase (APX) and catalase (CAT) in Beta vulgaris L. plants treated with combined EC50 Cd dose and different doses of Zn at 45 DAE. X-axis shows enzymatic antioxidants.
Figure 7.
Principal component analysis (PCA) showing the effect of cadmium (Cd) and zinc (Zn) on considered biochemical, physiological and yield parameters of spinach. The parameters studied are Total Chl (total chlorophyll), Chl a (chlorophyll a), Chl b (chlorophyll b), Caro (carotenoids), AsA (ascorbic acid), phenol, proline, APX (ascorbate peroxidase), SOD (superoxide dismutase), CAT (catalase), gs (stomatal conductance), Ps (rate of photosynthesis), Thiol, Protein, LPO (lipid peroxidation), H2O2 (hydrogen peroxide), and SOR (superoxide radical). Black dotted circles showed the synchronization of SOD and CAT.
The positive effects of Zn in Cd EC50 + Zn treated plants can be understood in light and dark reactions but also through increased concentration of chlorophyll contents. In the present study, increased chlorophyll contents in Cd EC50 + Zn treated plants were recorded compared to plants treated with CdEC50. Ma et al.,[51] also reported a considerable increase in chlorophyll content in wheat plants treated with Zn. Zn application not only insulates PSII from the detrimental effects of abiotic stress[52]; it also ensures the regular functioning of the different enzymes of dark reactions[53,54]. The present study indicated an ameliorated rate of photosynthesis in Cd EC50 + Zn plants, due to Zn compared to CdEC50 exposed plants, which further justifies the use of Zn in the management of Cd damage in plants.
-
The negative effects of CdEC50, as evident through the biomass and yield reduction, in the present study were improved by Zn application, with the maximum positive effect observed at 300 mg/L. The results suggest that Zn application is beneficial in improving the yield of spinach grown under Cd stress. Enhancement of yield upon Zn application can be attributed to the beneficial effect of Zn on the antioxidant activity which reduces the oxidative stress. Feedback of enzyme action showed that the SOD and CAT activity of plants treated with CdEC50 was more responsive to Zn compared to APX. The reduction in the thiol pool and inefficiency of the AsA-GSH cycle in CdEC50 + Zn treated plants depreciated their ascorbate regeneration potential, and enhanced the photosynthetic efficiency of plants. Biophysical characteristics responded positively to Zn amendment which led to improved carbon fixation efficiency of plants and resulted in increased yield of Cd EC50 + Zn treated plants compared to CdEC50 plants. Zn amendments improved membrane stability, enzymatic response, stomatal regulation, and photosynthetic yield of Cd treated plants, which resulted in enhancement of yield. The results of the present study justify the use of Zn as an efficient tool in the management of Cd toxicity in spinach plants. Zn (300 mg/L) diminished the CdEC50-induced oxidative activity by exhibiting antagonistic effects toward Cd toxicity. It is, however, necessary to establish a dose-response relationship to identify the appropriate functional dose of Zn, which does not show any synergistic effects with Cd toxicity.
The authors thank the Center of Advance studies, Department of Botany, for providing field and laboratory facilities and the Council of Scientific and Industrial Research (CSIR) and the University Grant Commission (USG) for financial support. Garima Yadav acknowledges CSIR and Parvati Madheshiya acknowledges UGC for their respective fellowships.
-
The authors confirm contribution to the paper as follows: study conception and design: Yadav G; data collection: Madheshiya P; analysis and interpretation of results: Madheshiya P; draft manuscript preparation: Tiwari S; All authors reviewed the results and approved the final version of the manuscript.
-
The datasets generated during and/or analyzed during the current study are available from the corresponding author on reasonable request.
-
The authors declare that they have no conflict of interest.
-
# Authors contributed equally: Garima Yadav, Parvati Madheshiya
- Copyright: © 2024 by the author(s). Published by Maximum Academic Press, Fayetteville, GA. This article is an open access article distributed under Creative Commons Attribution License (CC BY 4.0), visit https://creativecommons.org/licenses/by/4.0/.
-
About this article
Cite this article
Yadav G, Madheshiya P, Tiwari S. 2024. Boosting Beta vulgaris L. resistance to cadmium toxicity: the protective benefits of Zinc. Technology in Horticulture 4: e029 doi: 10.48130/tihort-0024-0024
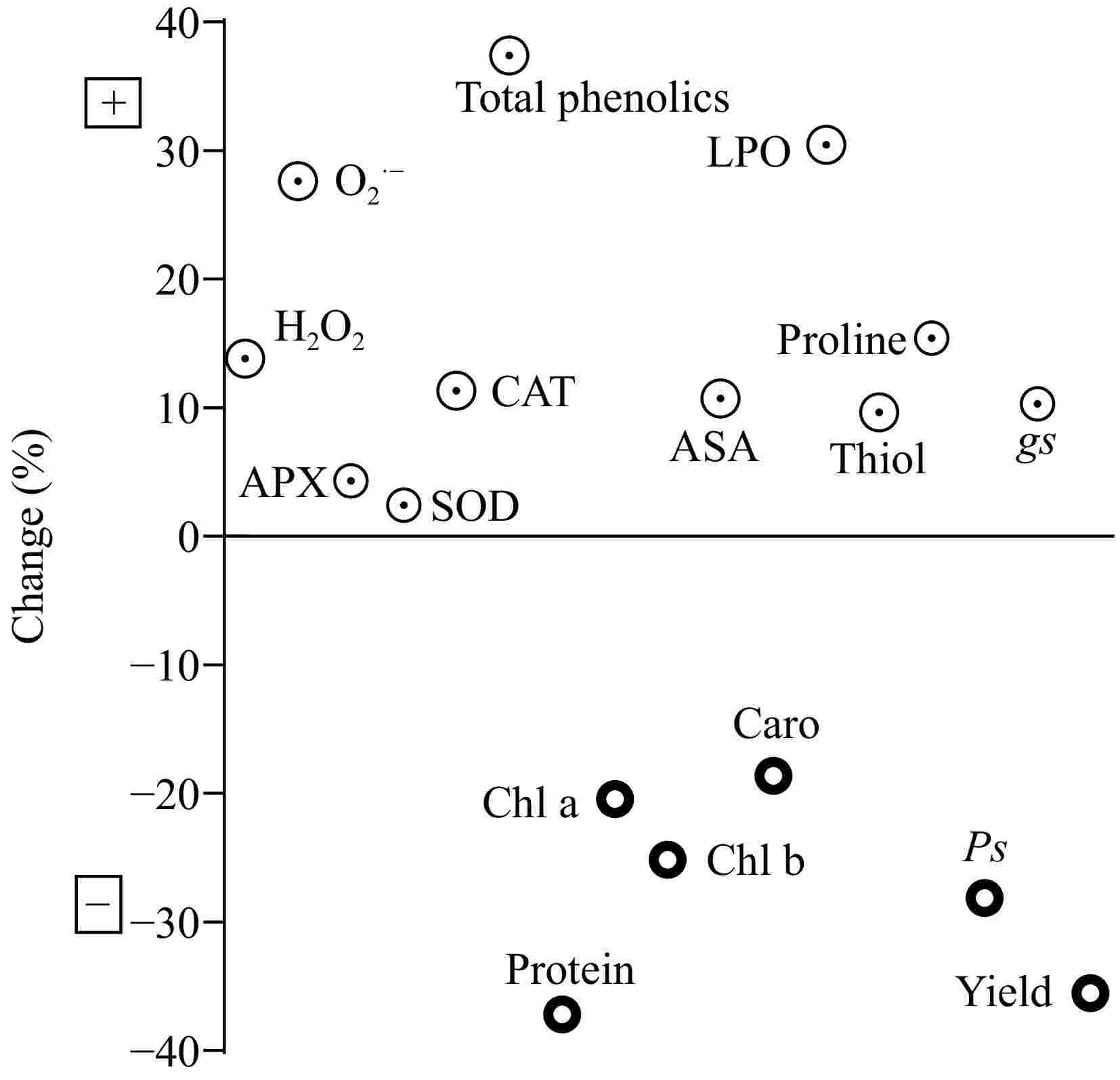