-
Tender coconut water (TCW) is derived from the liquid endosperm present in young, immature coconuts. While predominantly grown and consumed in the tropics, globally, TCW is recognized as a refreshing and energizing natural drink and also possesses a distinctive combination of micronutrients, phytohormones, and other bioactive compounds which makes it a functional drink with numerous health benefits[1]. Importantly, amidst increasing health consciousness among consumers, TCW is emerging as a healthy alternative to processed artificial and carbonated beverages.
Despite several health benefits and advantages, the utilization and marketability of TCW are limited owing to autolytic spoilage particularly due to PPO and POD. These enzymes result in browning, and oxidizing phenolic compounds, and contribute to off-flavor development significantly affecting the nutritional and sensory characteristics of TCW[2]. Thermal processing is the conventional approach widely used for enzyme inactivation nevertheless, leads to the degradation of quality parameters[3]. For instance, a study on pasteurization of TCW revealed that thermal treatment at 80 °C for 15 min resulted in reduced total soluble sugars, vitamin C, total phenolic content, and titratable acidity along with a reduction in sensory acceptability[4]. Another work, where TCW was treated at 136 °C for 8 s showed significant changes in the organic composition[5]. Considering the limitations of conventional preservation approaches, different novel thermal and non-thermal methods for preserving TCW are being explored and extensively studied by Naik et al.[6] and Prithviraj et al.[7]. With the increasing demand for TCW in different regions of the world, there is a rising focus on developing conveniently scalable approaches for TCW processing without the addition of chemicals/preservatives and the effects of high temperatures.
Utilizing various non-thermal methods such as ozonization, cold plasma technology, supercritical carbon dioxide, and high pressure processing can minimize spoilage and enhance the shelf life while maintaining the food quality. These novel approaches not only maintain food freshness but also preserve heat-sensitive nutrients within the food. Furthermore, these approaches act as excellent alternatives to conventional thermal processing while ensuring hygiene and safety, facilitating microbial decontamination, and better retention of nutritional and sensory characteristics. In addition, these approaches are considered to have a lower environmental impact[8]. Microfluidization is one such new and rapidly growing non-thermal processing technique in the food processing industry. Compared to the above-mentioned techniques, microfluidization offers uniform particle size reduction, increased scalability, and better energy efficiency with better flavor and color retention[9]. It involves pumping a liquid or semi-solid feed through a specifically designed interaction chamber (Y or Z type) at elevated pressures. Consequently, the feed experiences substantial shear, impact, and cavitation forces leading to particle breakdown and inducing various other physicochemical modifications[10]. Accordingly, microfluidization has gained multifaceted applications including encapsulation[11], extraction[12], modification of biological molecules[13], formation of stable emulsions/nanoemulsions[14], stabilization of plant-based milk, and so on.
In addition to the previously mentioned applications, microfluidization has been observed to exert a preservative effect by inactivating inherent enzymes and spoilage microbes. In a recent study conducted on sugar cane juice, notable findings revealed a substantial decrease in enzyme activity[15]. Another study conducted on sapodilla juice reported reducing activity of PPO from 100% to 80.78% and POD from 100% to 40.57%[16]. Further, a recent work involved the preservation of ginger rhizome juice by inactivating enzymes and microbes using microfluidization[17]. This indicated the suitability of microfluidization in the preservation and shelf life extension of natural fruit juices. However, so far, the application of microfluidization for the preservation of TCW has not been reported. Considering the enzymatic spoilage of TCW and heat sensitivity, microfluidization can emerge as an excellent processing technology providing high-quality clean label TCW. In this light, the current work aimed to evaluate the impact of microfluidization treatment on enzyme inactivation in TCW, whilst retaining key quality attributes. In this work, the TCW is treated at three different pressures and five levels of the number of passes/cycles, and the effect on the activity of key spoilage enzymes (PPO and POD) and other physicochemical attributes are assessed.
-
Tender coconuts (Cocos nucifera L.) of 5−7 months old maturity, Pollachi tall coconut variety were procured from a local farm in Thanjavur (10.7426° N, 79.1130° E), Tamil Nadu, India. The substrates for enzyme activity determination, namely pyrocatechol and guaiacol were procured from Himedia laboratories, Mumbai, India. All reagents and chemicals used in the determination of physicochemical properties were of analytical grade.
Collection of TCW
-
To avoid contamination, coconuts were disinfected by dipping in chlorinated water (~200 ppm chlorine) for 20 min followed by puncturing using a sterile stainless steel knife and collecting TCW under a sterile environment, which was further filtered using a muslin cloth to remove physical impurities. Care was taken to cause minimal damage to the tender coconut husk so that contamination was avoided.
Treatment of TCW
-
Freshly extracted TCW was treated in an laboratory-scale microfluidizer (LM20, Microfluidics, USA) equipped with a diamond interaction chamber (Y-type) at three different pressure levels [70 MPa (10,000 psi), 140 MPa (20,000 psi), and 210 MPa (30,000 psi)] and five different numbers of passes/cycles (3, 5, 7, 9, and 11). The amount of TCW sample taken for each experimental run was 250 mL. The choice of pressure conditions was dependent on the operating conditions of the microfluidizer equipment and previous research reports. The number of passes were fixed based on the preliminary experiments on the enzymatic activity. To prevent the temperature increase, the cooling coil was kept submerged in a cold temperature water bath (15 ± 2 °C).
Determination of enzymatic activity
PPO activity
-
The activity of PPO was determined according to the method adopted by Campos et al.[2] with minor variations. A 5 mL phosphate buffer (0.2 M, pH 6.0) and 1.5 mL pyrocatechol solution (0.2 M) were taken in a test tube and kept at 25 °C in a water bath (LMWB-04, Labman Scientific Instruments, Chennai, India) for 10 min followed by the addition of 1 mL TCW sample. The contents were mixed well and the absorbance was recorded every minute at 425 nm for 30 min using a multi-mode reader. A mixture of 5.5 mL phosphate buffer and 1.5 mL pyrocatechol solution was treated as blank. The activity of PPO was determined according to Equation (1).
Enzymeactivity=K0.001×DF (1) where, DF is the dilution factor of TCW (~6), and K represents the slope of the line obtained by plotting the absorbance values against time.
The experiment was repeated three times and enzymatic activity was calculated according to Equation (1). The PPO activity was measured in units of enzyme activity (U/mL), where one unit is defined as the amount of enzyme required to cause a change of 0.001 in absorbance per minute.
To compare the influence of microfluidization treatment, the resulting values are expressed in terms of the percentage reduction in the enzyme activity and calculated according to Equation (2)[18].
%Reductioninenzymeactivity=PPOc−PPOmPPOc×100 (2) where, PPOc and PPOm indicate the PPO activity in untreated (control) and microfluidized TCW samples.
POD activity
-
POD activity was measured by adopting the procedure followed by Fehrmann & Diamond[19]. Briefly, a test tube containing 1 mL TCW and 7 mL of 0.2 M phosphate buffer (pH 5.5) was placed in a water bath at 35 °C for 10 min. Afterward, 1.5 mL of 0.05% guaiacol and 0.5 mL of 0.1% hydrogen peroxide were added, and absorbance was noted every minute at 470 nm with a multi-mode reader (Spectra Max iD3, Molecular Devices LLC, USA). POD activity was calculated using Equation (1), where the DF represents the dilution factor of TCW (~5) and percentage reduction was measured using Equation (2). The measurement of POD activity was indicated in units of enzyme activity (U/mL) where one unit (U) of enzyme activity is equivalent to the amount of enzyme responsible for a 0.001 change in absorbance for every minute.
Modeling enzyme inactivation
-
Enzyme inactivation kinetics helps in an adequate understanding of the process and optimization of process parameters. To model the inactivation kinetics, the residual activity of the enzyme was calculated according to Equation (3).
Residualactivity=AtA0×100 (3) where, At and A0 represent the enzyme activity of microfluidized and controlled TCW samples. The experimental data of the enzyme activity was modeled using zero-order and first-order kinetics models according to Equations (4) and (5), respectively[20].
RAn=RA0−kn (4) RAn=RA0×e−kn (5) where, RAn and RA0 represent the residual activity of the enzyme of the sample treated at the nth pass and the control sample, respectively. The rate constant is represented by k and n is the number of passes.
Quality evaluation of TCW
Color
-
The color of control and treated TCW samples was measured using a Hunter lab colorimeter (Colorflex, EZ 45/0-LAV, Hunter Associates Laboratory Inc., USA). A 5 mL sample was placed in a sample holder and the measurements were recorded. L* represents lightness, a* represents redness to greenness, and b* value represents the yellowness to blueness range. The color difference (ΔE) between the control and treated TCW was computed using Equation (6).
ΔE=√ΔL∗2+Δa∗2+Δb∗2 (6) Turbidity
-
The turbidity of TCW was analyzed by measuring the absorbance at 610 nm using the multi-mode reader with distilled water as a blank[2]. Therefore, the transmittance and turbidity (%) were determined using Equations (7) and (8), respectively.
Transmittance=100×(10−abs) (7) Turbidity=100−Transmittance (8) where, abs is the absorbance at 630 nm.
Total soluble solids
-
The total soluble solids (TSS) content of TCW was determined using a handheld refractometer at room temperature (Bombey Scientific, Erma Make, Kanpur, India; measurement range: 0−32 °Brix) and expressed in °Brix.
pH and titratable acidity
-
The pH of control and treated TCW samples was measured using a pH meter (PC-700, EU tech Instruments, Thermo Fisher Scientific Pvt Ltd, India; accuracy ± 0.01). For determination of titratable acidity, (TA) 5 mL TCW was transferred into an Erlenmeyer flask with the addition of 100 mL distilled water and titrated against NaOH (0.1 N) till the appearance of a faint pink color that lasted for 30 s. TA was calculated and expressed in an equivalent weight of % malic acid (w/v)[21] using Equation (9).
TA=N×M×VVc×100 (9) where, V is the volume of NaOH, N is the normality of NaOH, M is the malic acid factor (67.05) and Vc indicates the volume of TCW added.
Protein content
-
The protein content was assessed by using the method given by Lowry et al.[22]. In a test tube, 1 mL TCW sample and 5 mL of alkaline CuSO4 solution were added. The contents were incubated at room temperature for 10−15 min. A 0.5 mL of Folin-Ciocalteau reagent was added, and mixed vigorously, followed by incubation for 30 min. Absorbance was read at 660 nm using a multi-mode reader. The procedure was repeated with 1 mL of distilled water as blank. The protein content was determined by using a standard curve obtained with bovine serum albumin as a standard.
Total sugar content
-
Total sugars in the samples were estimated using the AOAC standard method[23]. A 0.1 mL of TCW was diluted with distilled water to a total volume of 1 mL. To this solution, 4 mL anthrone reagent (200 mg anthrone in 100 mL of 95 g/L sulfuric acid) was added and the contents were incubated in a boiling water bath for 8 min. The test tubes were cooled rapidly and the absorbance was measured at 630 nm using a multi-mode reader. The total sugar content was calculated by comparing the results with a standard glucose curve.
Total phenolic content
-
The total phenolic content (TPC) of TCW was estimated using a colorimetric method reported by Alothman et al.[24] with minor modifications. A 1 mL TCW sample was mixed with 1.5 mL of Folin-Ciocalteau reagent (10 times diluted) and incubated at room temperature for 5 min. Further, 4 mL of Na2CO3 solution (3.5%) was added and again incubated for 30 min. The absorbance was measured at 700 nm using a multi-mode reader. TPC was quantified using a gallic acid standard curve and expressed as milligram gallic acid equivalents per mL (mg GAE/mL).
Total flavonoid content
-
The total flavonoid content (TFC) of the samples was analyzed following the method outlined by Xu & Chang[25] with slight modifications. Samples were appropriately diluted to 1 mL and 0.3 mL NaNO2 (5%) was added to it. After 5 min of incubation, 3 mL AlCl3 (10%) was added, followed by 2 mL NaOH (1 M). Absorbance at 510 nm was noted and TFC was determined as milligrams of quercetin equivalents (QE) per gram of defatted sample using a standard curve.
Antioxidant activity
-
The antioxidant activity of the TCW samples was estimated using the 2,2-diphenyl-1-picrylhydrazyl (DPPH) assay as reported by Brand-Williams et al.[26] and Bursal & Gülçin[27]. To prepare the stock solution, 24 mg of DPPH was dissolved in 100 mL of methanol and stored at 20 °C until required. The working solution was prepared by diluting the DPPH stock solution with methanol to achieve an absorbance of 0.98 ± 0.02 at 517 nm using a spectrophotometer. A 3 mL portion of this solution was then mixed with 100 μL of the TCW at various concentrations (10–500 μg/mL). The reaction mixture was thoroughly shaken and incubated in the dark at room temperature for 15 min. Absorbance was subsequently measured at 517 nm with methanol as blank. For control methanol was used instead of the sample and the absorbance was noted. The radical scavenging activity was calculated according to Equation (10).
Radicalscavengingactivity(%)=(Controlabsorbance−Sampleabsorbance)Controlabsorbance×100 (10) Statistical analysis
-
All experiments were conducted in triplicate and the results are presented as the mean value ± standard deviation. All obtained results were subjected to a two-way analysis of variance (ANOVA) with Duncan's test to observe significant differences within experimental variables at a 5% significance level using SPSS (ver. 20, IBM Corporation, USA).
-
PPO is primarily responsible for the discoloration of TCW[28]. Figure 1 shows the sample before and after the treatment. The impact of microfluidization treatment on the activity of PPO is reported in Fig. 2a. It was evident that microfluidization was not able to entirely inactivate PPO, but a substantial reduction (p < 0.05) was observed. At lower pressures of 70 and 140 MPa, no significant differences in three and five passes. However, at 210 MPa, with an increasing number of passes, a higher reduction in PPO activity was evident. Nonetheless, after the 9th cycle, no further significant differences were noted. The highest reduction in enzyme activity was recorded at 210 MPa pressure after the 11th pass (~61%).
Figure 1.
Images of control (without microfluidization treatment) and microfludized TCW samples, showing insignificant visual changes.
Reduction in enzymatic activity can be ascribed to the irreversible conformational changes in the tertiary and quaternary structure of enzymes. Partial unfolding and molecular rearrangements lead to the generation of less functional or non-functional active sites due to which the enzyme activity is reduced. The most significant reduction in PPO activity was witnessed when applying a pressure of 210 MPa with 11 passes through the system. These results are comparable with work performed by Tarafdar et al.[18] wherein a maximum reduction of PPO activity (64.7 %) in sugarcane juice was observed at 150 MPa pressure in a single pass. The effect of microfluidization treatment on PPO activity strongly depends on the matrix of the sample. Increased microfluidization pressures reduce treatment time by accelerating the pumping of juice through the interaction chamber, potentially leading to insufficient enzyme deactivation compared to other conditions, suggesting the need for engineering modifications to extend the holding time. In a recent study by Singh et al.[16] on the microfluidization of sapodilla juice, a notable increase in PPO activity was observed following the microfluidization treatment. Another work on mushroom[29] is also in agreement with these findings. In addition to differences in composition/matrix, such variations can be attributed to the source and conformation of PPO. Decreased enzyme activity may result from alterations in tertiary and quaternary enzyme structures, which occur owing to the disruption of non-covalent hydrogen bonds[30]. Balny & Masson[31] investigated that the impact of multiple passes was notably significant (p < 0.05), causing an additional 6%–17% decrease in PPO activity, particularly at elevated pressure levels, with the most significant reduction observed at 140 MPa. Multiple passes could potentially disturb the electrostatic and hydrophobic interactions within the enzyme structure, resulting in its inactivation.
Effect on POD
-
POD belongs to the group of oxidoreductases that can catalyze polyphenolic oxidation[2,21] and is also responsible for producing off-odors. From Fig. 2b, contrary to its effects on PPO, it was observed that the percentage reduction of POD was higher when treated at 70 MPa (that is, a 45% reduction at the 9th pass), as compared with TCW treated at 140 and 210 MPa microfluidization pressures. The least reduction in the enzyme activity was perceived in the case of 140 MPa. These results follow a similar trend as in the study reported by Tarafdar et al.[15] in sugar cane juice wherein the authors reported a higher reduction in POD activity at 50 MPa and the lowest reduction at 200 MPa. It is expected that these trends are resulting from the effect of the treatment time. Higher microfluidization pressures shorten treatment times by accelerating the pumping of TCW through the interaction chamber. However, lower holding time may not be sufficient to achieve the desired level of enzyme inactivation. Further, the present work explains that the inactivation of POD in TCW requires less intense conditions as compared to PPO and this can be attributed to the structure of these enzymes. From a different point of view, a work on thermal inactivation of PPO and POD also revealed that PPO is more thermostable[21]. The study reported by Terefe et al.[32] revealed that treatment time plays a significant impact on POD inactivation in strawberry puree. Similarly, in the present study, the longer holding time in 9 passes at 70 MPa shows a reduction in POD activity. As suggested by Balny & Masson[31], during the initial stages of thermal inactivation, proteins undergo the loss of crucial water molecules, potentially leading to structural rearrangement. Conversely, high pressure could impede this phenomenon by enhancing the hydration of both charged and non-polar groups. Pressure increases (for POD) can result in protein unfolding and alterations in protein hydration, consequently resulting in the apparent loss of enzyme activity due to protein denaturation. The denaturation pressure threshold may vary depending on the specific protein or enzyme[33].
Inactivation kinetics of PPO and POD
-
The enzyme inactivation kinetics modeling plays a vital role in the optimization of conditions, process design, scale-up, and industrial implementation of technology[34]. The inactivation kinetics of PPO and POD as a result of microfluidization pressure and a number of passes were modeled using zero-order and first-order kinetics models. The model parameters and coefficient of determination (R2) are stated in Table 1. It can be observed that the suitability of the model for predicting the enzyme inactivation strongly depended on the microfluidization pressure. At 70 MPa pressure, both models failed to adequately represent the inactivation behaviour of PPO and POD. However, both models showed higher R2 values at 140 and 210 MPa pressure. The dependence of the model fitting on experimental conditions has been previously reported. Bai et al.[35] reported that the model fit of inactivation kinetics of PPO in Fuji apple depended on the blanching temperature. At a temperature lower than 100 °C, the zero-order kinetics model was suitable whereas, above 100 °C, the first-order kinetics model was found to adequately represent the inactivation behavior.
Table 1. PPO and POD inactivation kinetic parameters and coefficient of determination (R2) of zero-order and first-order models at different microfluidization pressures.
Model Pressure
(MPa)PPO POD k (pass−1) R2 k (pass−1) R2 Zero order 70 3.0079 ± 0.2043 0.48 4.0982 ± 0.2052 0.65 140 3.1576 ± 0.2266 0.89 2.4915 ± 0.1483 0.84 210 6.085 ± 0.1999 0.96 2.2642 ± 0.1068 0.99 First order 70 0.0352 ± 0.0026 0.49 0.0518 ± 0.0034 0.64 140 0.0359 ± 0.0028 0.88 0.0294 ± 0.0014 0.85 210 0.0866 ± 0.0037 0.96 0.0252 ± 0.0013 0.99 According to the zero-order kinetics model, in case of PPO, the rate constant showed a ~2-fold increase as pressure increased from 70 to 210 MPa. On the other hand, the first-order kinetics model rate constant exhibited a nearly 2.5-fold increase when pressure rises above the said range. This suggests that PPO inactivation is accelerated at higher microfluidization pressure. Similarly in another work on ultra-high pressure treatment of Litopenaeus vannamei, as the pressure increased, the inactivation of PPO also increased[20]. However, the opposite trend was noted in the case of POD inactivation, where both models showed greater values of rate constants at 70 MPa pressure. These opposite trends can be attributed to the complexities associated with the structural and conformational changes in enzymes as a result of multiple acting forces during microfluidization. Based on the findings from previous studies[31], the denaturation of PPO and POD enzymes occurs exclusively under exceptionally high-pressure conditions. The present study supports this hypothesis, indicating that activation effects perceived during moderate pressure treatments are likely due to reversible conformational changes of enzyme or substrate molecules. This indicates that the application of pressure may lead to changes in enzyme structure without causing irreversible damage, as supported by the pH dependency of these activation effects.
Effect of microfluidization on physicochemical properties
-
All processing technologies impact the quality of food products in numerous ways. An ideal processing technology should exert minimal impact on the quality of processed food. This section elaborates on the effect of microfluidization on the key features of TCW.
Color
-
Color is an important parameter for the consumer acceptability of beverages and the preservation of natural colors during processing and storage is a challenge. In this work, the impact of microfluidization on the color of TCW is presented in terms of L*, a*, b*, and color difference (ΔE) and reported in Table 2. It can be perceived that the pressure is a more significant factor affecting the color compared to the number of passes. The lightness value showed an increasing trend with increasing pressure, whereas treatment at 210 MPa showed the highest increase in the L* value. These results are in agreement with increasing turbidity value. The a* value did not show any significant trend whereas the b* value showed an inverse relation with pressure and number of passes. Furthermore, it has to be noted that the PPO enzyme which is responsible for browning reactions is effectively being inactivated by microfluidization helps prevent noticeable colour changes. This is reflected in ΔE where it demonstrated a direct relation with treatment intensity (pressure and number of passes). It is regarded that ΔE values less than 2 imply that color changes are visually unnoticeable[36] as can be observed in in Fig. 1. At 70 and 140 MPa pressure, ΔE value ranged between 0.26 to 2.42, explaining a minimal impact of the process on the color. At 210 MPa, a drastic rise in ΔE value was noticed for samples treated for seven and 11 passes. Similar finding was also reported by Tarafdar et al.[37] during the microfluidization of sugarcane juice at 140 MPa (~20,000 psi). The increase in ΔE values can also be associated with the destruction of cellular components due to the increment in pressure which can release polyphenol compounds which is significant to the improved quality of TCW after microfluidization.
Table 2. Effect of microfluidization on physicochemical properties of TCW.
Sample Color (ΔE) L* a* b* Turbidity (%) TSS (°Brix) pH TA (%) Control/untreated TCW NA 5.770 ± 0.670Aa −0.320 ± 0.05Aa −1.970 ± 0.060Aa 2.59 ± 0.14Aa 5.20 ± 0.00Aa 5.50 ± 0.01Aa 0.06 ± 0.00Aa Pressure (MPa) No. of passes/cycles 70 3 0.26 ± 0.025Aa 6.035 ± 0.007Bb −0.335 ± 0.106Ab −1.745 ± 0.035Ba 3.22 ± 0.28Ba 5.05 ± 0.40Aa 5.50 ± 0.01Ab 0.06 ± 0.004Aa 5 0.34 ± 0.017Ac 6.465 ± 0.035Bb −0.335 ± 0.035Ac −2.210 ± 0.041Bb 3.54 ± 0.20Bb 5.00 ± 0.04Aa 5.51 ± 0.01Ab 0.05 ± 0.006Aa 7 0.55 ± 0.004Ab 6.390 ± 0.028Bc −0.330 ± 0.014Abc −2.165 ± 0.035Bcf 5.31 ± 0.25Bc 5.01 ± 0.23Aa 5.49 ± 0.01Aab 0.05 ± 0.001Aa 9 0.61 ± 0.011Ab 6.565 ± 0.021Bd −0.375 ± 0.007Ac −2.180 ± 0.042Bdf 6.19 ± 0.03Bd 5.00 ± 0.32Aa 5.50 ± 0.00Aab 0.04 ± 0.001Aa 11 0.72 ± 0.032Ad 7.480 ± 0.042Be −0.385 ± 0.007Abc −2.230 ± 0.057Be 6.24 ± 0.06Be 5.00 ± 0.39Aa 5.47 ± 0.02Aa 0.05 ± 0.001Aa 140 3 0.92 ± 0.033Ba 5.660 ± 0.014Ab −0.335 ± 0.106Bb −1.610 ± 0.077Ca 3.77 ± 0.11Ca 5.03 ± 0.04Aa 5.55 ± 0.06Ab 0.05 ± 0.004Aca 5 1.46 ± 0.044Bc 6.110 ± 0.007Ab −0.500 ± 0.035Bc −1.740 ± 0.007Cb 4.93 ± 0.08Cb 5.01 ± 0.01Aa 5.51 ± 0.00Ab 0.05 ± 0.003Aca 7 1.55 ± 0.019Bb 6.740 ± 0.021Ac −0.430 ± 0.007Bbc −1.680 ± 0.014Ccf 5.02 ± 0.06Cc 5.01 ± 0.01Aa 5.48 ± 0.01Aab 0.05 ± 0.006Aca 9 1.61 ± 0.010Bb 5.900 ± 0.014Ad −0.410 ± 0.021Bc −1.590 ± 0.014Cdf 5.28 ± 0.03Cd 5.00 ± 0.00Aa 5.47 ± 0.03Aab 0.04 ± 0.010Aca 11 2.42 ± 0.015Bd 6.090 ± 0.028Ae −0.320 ± 0.021Bbc −1.800 ± 0.014Ce 7.22 ± 0.08Ce 5.00 ± 0.00Aa 5.45 ± 0.03Aa 0.04 ± 0.013Aca 210 3 1.59 ± 0.221Ba 15.870 ± 0.537Cb −0.490 ± 0.014Cb 0.460 ± 0.014Da 4.23 ± 0.16Da 5.43 ± 0.53Aa 5.52 ± 0.01Bb 0.03 ± 0.030BCa 5 2.43 ± 0.521Bc 15.020 ± 0.255Cb −0.550 ± 0.014Cc −0.400 ± 0.071Db 5.64 ± 0.07Db 5.30 ± 0.42Aa 5.51 ± 0.01Bb 0.03 ± 0.028BCa 7 2.98 ± 0.031Bb 10.460 ± 0.070Cc −0.610 ± 0.007Cbc −0.770 ± 0.028Dcf 6.88 ± 0.15Dc 5.41 ± 0.56Aa 5.48 ± 0.01Bab 0.03 ± 0.028BCa 9 3.16 ± 0.076Bb 9.010 ± 0.007Cd −0.620 ± 0.028Cc −0.960 ± 0.021Ddf 7.41 ± 0.13Dd 5.40 ± 0.57Aa 5.49 ± 0.00Bab 0.02 ± 0.023BCa 11 4.61 ± 0.018Bd 10.680 ± 0.028Ce −0.620 ± 0.021Cbc −0.880 ± 0.014De 8.62 ± 0.39De 5.35 ± 0.49Aa 5.46 ± 0.00Ba 0.02 ± 0.026BCa Uppercase letters (A, B and C) and lowercase letters (a, b, c, d, and e) indicate significant differences between pressure and number of passes/cycles, respectively, at a 5% level of significance (p < 0.05). Turbidity
-
Turbidity explains how much a solution is opaque due to the presence of suspended contents. TCW is considered a natural drink that is clear and transparent in appearance. The increase in turbidity may impart cloudiness and will have a significant impact on consumer acceptability. The changes in turbidity as a result of microfluidization are presented in Table 2. A significantly increasing relationship (p < 0.05) between the number of passes, and pressures and turbidity was observed. A value of 2.59% ± 0.10% was seen in the case of the control sample and the highest value (8.62% ± 0.39%) was observed for TCW treated at 210 MPa for 11 passes. An increase in the turbidity of TCW can be attributed to the cell disintegration taking place due to microfluidization, in turn, releasing some cellular components that contribute to the turbid nature of the TCW[38]. Nevertheless, the cellular disintegration in microfluidization does not have any negative implications on product quality and shelf life.
Total soluble solids
-
Total soluble solids (TSS) are linked with the maturity stage and sweetness of the TCW. Typically, the TSS of TCW range from around 5 to 5.6 °Brix[21]. As shown in Table 2, it was observed that there were no differences in TSS due to the number of passes and pressures. This observation suggests that the conversion of non-reducing sugars into reducing sugars through hydrolysis or inversion is not taking place[39]. In addition, Tan et al.[21] stated that the levels of non-reducing sugars are lower in TCW and increase with maturity.
pH
-
Typically, pH affects enzyme activity, charge distribution, and enzyme-substate binding. Further, Klomklao et al.[40] reported that extremely acidic and alkaline pH conditions may result in irreversible enzyme denaturation. The pH of all samples ranged between 5.46 to 5.51 (Table 2). At lower pressures (70 and 140 MPa) there were insignificant variations in pH (p > 0.05). However, at 210 MPa, a slight reduction in the pH was observed with an increasing number of passes. It is considered that the microfluidization is responsible for causing localized heating of the product[15]. A reduction in the pH can be attributed to localized temperature rise resulting in the conversion of sugars to acids occurring at higher pressure. Pandiselvam et al.[41] also reported similar results with a reduction in pH from 5.2 to 5.03 during microwave treatment of tender coconut water. As in Table 2, TA did not change with the number of passes. However, at 140 and 210 MPa pressures, a slight change was observed. To better understand the underlying mechanisms, there is a need for future work to clearly explain the individual and combined effects of shear, cavitation, and viscous dissipation on different foods and their quality parameters.
Protein content of TCW
-
The protein content remained almost similar in the control and treated samples (Fig. 3a). Though proteins may undergo modification in the microchannels during the treatment process, TCW has a protein content of ≤ 1 mg/mL and these effects were not very evident. Earlier, it has been reported that microfluidization can alter the protein structure, for instance, of cowpea flour, improving its physical properties[42].
Figure 3.
(a) Protein content, and (b) total sugar content of control and microfluidized TCW samples.
Total sugar content
-
The total sugar content of TCW showed an increase upon treatment with microfluidization (Fig. 3b). Treatment at 70 and 140 MPa showed a significant rise in total sugar content compared to control (p < 0.05). The tendency is reported with an increasing number of passes up to five passes after which the rise was insignificant. These changes can be accredited to the hydrolysis of carbohydrates and starch into simple sugars[43]. In a work on microfluidization of sugarcane juice, reducing sugar concentration increased compared to the untreated sample[18].
Total phenolic content
-
The total phenolic content of the control and microfluidized TCW samples are reported in Fig. 4a. It can be observed that microfluidization pressure is a significant factor affecting the TPC compared to the number of passes. Samples treated at 70 MPa reported an increase in the TPC relative to the control sample (p < 0.05). Similar results are reported in an Ottoman strawberry juice where treatment at 100 MPa significantly increased the TPC content. An increase in the TPC can be ascribed to the breakdown of cellular components releasing phenolic compounds and corresponding decay of the deteriorating enzymes such as PPO and POD[44]. However, this trend is not followed by a further increase in pressure. Both samples treated at 140 and 210 MPa showed no significant difference from the control. Furthermore, it is also essential to note that at higher pressure localized heating of the product may take place resulting in thermal loss of phenolic compounds which is observed at 140 MPa pressure. Furthermore, there is a slight increase in the phenolic content at 210 MPa. In microfluidization at higher pressure, the residence time of samples is low. Due to comparatively lower residence time, the sample might have shown better retention of released phenolic compounds compared to the one treated at 140 MPa. Pertaining to these intricate interactions, the TPC content did not follow any specific trend with respect to pressure and number of passes. Similarly, Koley et al.[45] did not observe any clear trend in the TPC of microfluidized carrot juice.
Figure 4.
(a) Total phenolic content (TPC), (b) total flavonoid content (TFC), and (c) % radical scavenging activity (RSA) of control and microfluidized TCW samples.
Total flavonoid content
-
TFC in control was determined as 0.409 mg QE/ml as compared to 0.224–0.304 mg QE/ml in microfluidized tender coconut water (Fig. 4b). Flavonoids are a class of polyphenolic secondary metabolites that can correlate with antioxidant activity[46]. Here, the flavonoids showed higher degradation compared to the phenolic compounds indicating their susceptibility to high pressure and cavitation during microfluidization. A work on TCW by Rajashri et al.[47] also reported higher losses of flavonoids than phenolic compounds as a result of ultrasound treatment which also works on the principle of cavitation. The results indicated that decrease in flavonoid content at higher microfluidization pressure whereas, no remarkable difference in the flavonoid content among various passes which is in agreement with Tarafdar et al.[37]. Localized heat generation as a result of intense pressure could possibly decrease in the TFC[15].
Antioxidant activity
-
The antioxidant activity of treated and untreated tender coconut water is depicted in Fig. 4c. It can be observed that there is a slight increase in the antioxidant activity of treated samples in comparison to the control sample. During microfluidization, intense forces lead to the breakdown of cellular components leaching active ingredients. These results are in agreement with the rise in TPC of treated TCW samples. Comparable findings were reported in a work on the microfluidization of peach juice where a 16% increase in total AA was observed[48]. Dynamic high pressure microfluidization (DHPM) processing may stimulate the release of active ingredients in fruits, potentially enhancing antioxidant activity. High pressure can modify the tissue matrix by disrupting cell walls, which could lead to the release of antioxidant compounds into the extracellular environment[49]. Velázquez-Estrada et al.[50] utilized ultra-high pressure homogenization to process orange juice and assessed changes in antioxidant activity using the FRAP method. They revealed that applying high pressure homogenization between 100−300 MPa significantly reduced the antioxidant activity in orange juice. Interestingly, no significant differences were observed among pressures of 100, 200, and 300 MPa. Wang et al.[48] investigated that the DHPM at 20 MPa for a single pass showed no significant impact on antioxidant activity. As pressure increased, the DHPM processing effectively preserved antioxidant activity.
-
Microfluidization was observed to substantially reduce the enzyme activity of PPO and POD in TCW. PPO showed better inactivation at higher pressure (210 MPa); whereas, POD was found to exhibit minimum residual activity at relatively lower pressure (70 MPa). Thus, it is concluded that moderate pressure with a greater number of passes can achieve effective inactivation of both enzymes. Enzyme inactivation kinetics also revealed the dependence of the rate constant on the pressure during microfluidization. This study reports that the microfluidization treatment exerted a minimal impact on the physicochemical properties of TCW. It was also observed that flavonoids are more susceptible to microfluidization treatment compared to phenolic compounds. A minor degradation of these compounds could be linked to slight thermal impact at intense processing conditions which can be overcome by precooling the sample before the treatment. Microfluidization as a standalone treatment could not achieve complete inactivation of enzymes, nevertheless, using hybrid treatment approaches will provide synergistic effect in achieving complete inactivation of spoilage enzymes. Furthermore, future research has to be directed toward studying the effect of microfluidization on spoilage microorganisms in TCW thereby further improving the shelf life.
-
The authors confirm contribution to the paper as follows: study conception and design: Moses JA, Sinija VR; data collection: Sruthi PS, Vanmathi Mugasundari A; analysis and interpretation of results: Sruthi PS, Vanmathi Mugasundari A, Nimbkar S; draft manuscript preparation: Sruthi PS, Vanmathi Mugasundari A, Nimbkar S; supervision: Moses JA. All authors reviewed the results and endorsed the final version of the manuscript.
-
All data produced or analyzed in this study are incorporated within this manuscript.
This research did not receive funding from any specific grant provided by public, commercial, or not-for-profit sectors.
-
The authors declare that they have no conflict of interest.
-
Authors contributed equally: P. S. Sruthi, A. Vanmathi Mugasundari
- Copyright: © 2024 by the author(s). Published by Maximum Academic Press on behalf of China Agricultural University, Zhejiang University and Shenyang Agricultural University. This article is an open access article distributed under Creative Commons Attribution License (CC BY 4.0), visit https://creativecommons.org/licenses/by/4.0/.
-
About this article
Cite this article
Sruthi PS, Vanmathi Mugasundari A, Nimbkar S, Moses JA, Sinija VR. 2024. Microfluidization of tender coconut water and its impact on spoilage enzymes and physicochemical properties. Food Innovation and Advances 3(4): 344−352 doi: 10.48130/fia-0024-0030
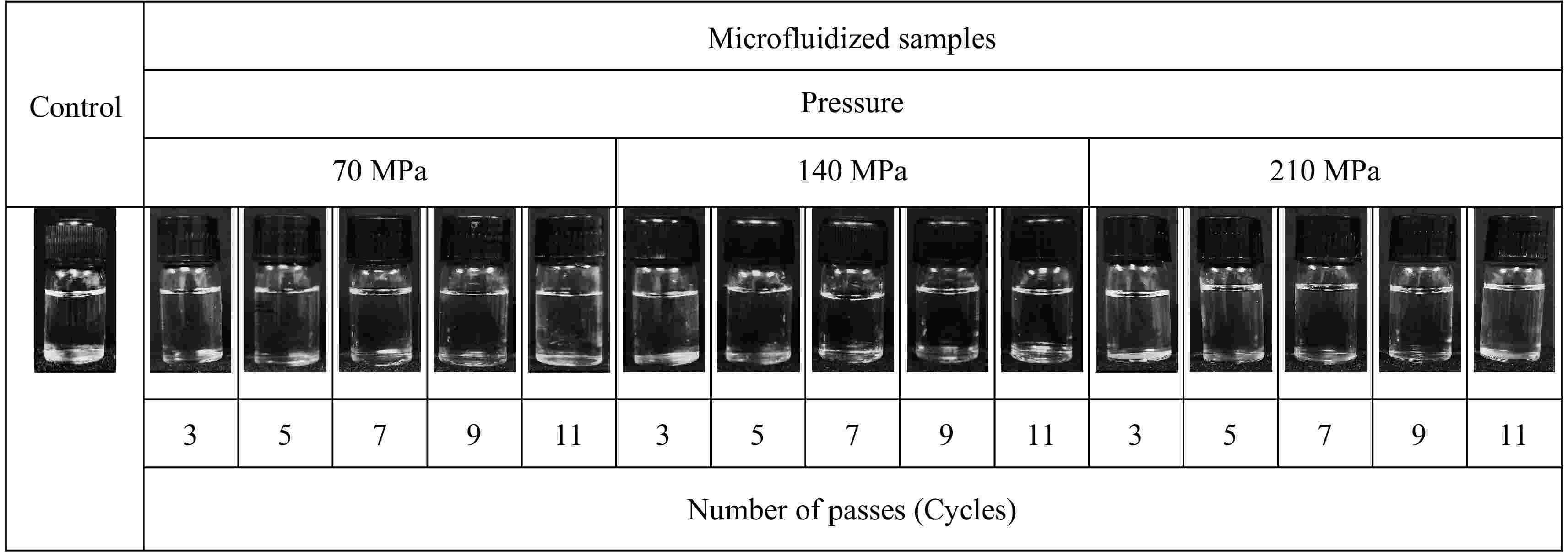