-
Traditionally, fish is considered an affordable source of protein of high biological value, including high levels of essential amino acids (lysine, methionine, etc.), lipid-soluble vitamins, minerals (Se, P, Fe, Mg, and K), and rich in highly unsaturated fatty acids (ω3, 6, and 9), such as docosahexaenoic and eicosapentaenoic acid[1, 2]. The lipid fraction and other bioactive components present in fish, have attracted a great deal of attention because of their favorable effects on human health[3], including reducing the risk related to human cardiovascular and chronic neurodegenerative diseases. Accordingly, the World Health Organization and American Heart Association have also recommended consuming 1−2 servings of fish weekly regularly. Nevertheless, quality preservation of fish and fish products is challenging due to their high perishability. Further, due to high water and non-protein nitrogen content, the freshness of post-mortem fish muscle rapidly declines. The deterioration in quality of fish due to spoilage by microorganisms[4]. Further microbial spoilage leads to lipid oxidation resulting deterioration of different quality aspects of fish. In general, fish spoilage is governed by three basic mechanisms namely enzymatic autolysis, microbial growth and lipid peroxidation. All three, in a favourable condition continue simultaneously in a food substrate and after a certain interval, the evidence of spoilage could be noticed.
Spoilage is considered as one of the major concerns of fish food safety which may cause several negative effects on the health of the consumers. For this reason, commonly, one or more preservation technique(s) is employed to extend their shelf life, with the purpose to prevent and or delay quality changes[5]. Traditional preservation methods, including drying by different means such as salting, smoking, fermentation, etc., low-temperature storage (chilling, freezing), or with chemical preservatives, have been commonly used in the industry. Nowadays, non-thermal physical technologies (pulsed electric fields-PEF, high hydrostatic pressure-HPP, ionizing radiation ultrasonication, cold plasma, and innovative packaging systems) are also being employed for the same purposes[6]. Likewise, PEF and HHP treatments may not ensure food safety, as these can only cause sublethal damage to the bacterial cell wall[7]. Beside processing strategies, various organic acids and their different salts (such as sorbic acid, benzoic acid, propionic acid, sodium benzoates, propionates, potassium sorbates, nitrites, ascorbic acid, citric acid, etc.), and synthetic phenolic compounds such as butylated hydroxyl toluene (BHT), butylated hydroxyl anisole (BHA), tert-butylated hydroquinone (TBHQ), dodecyl gallate are used as food preservatives[8]. Incorporating synthetic antioxidants viz., BHT, BHA, TBHQ at high doses, either to the raw materials or end-products can negatively affect human health[9]. Prolonged use of these synthetic preservatives and compounds are reported to induce cancer, liver and kidney damage, gastrointestinal disorders, asthma, and many allergies[10].
With increased concerns and negative perceptions among consumers regarding the safety aspects of chemical preservatives and synthetic compounds, the demand for minimally processed ready-to-eat fish products has increased many-fold. To cater to the demand of consumers and to ensure the availability of safe, nutritious, tasty, and convenient food, natural alternatives, such as those involving phytochemicals/phytoextracts, including essential oils, phenolics etc. derived from plants have gained lots of interest. Plants and their parts harbor a complex mixture of bioactive compounds that are a good source of phytochemicals such as polyphenols, phytosterols, alkaloids, nitrogen-containing compounds, terpenoids, organosulfur compounds etc. These compounds possess inherent biological effects such as antimicrobial, antioxidant, antidiabetic, anti-inflammatory, immune-enhancing functions etc. that are reported to offer health benefits[11−13] and form the major basis of Ayurveda, Unani, Siddha and the Chinese system of medicine since antiquity.
As the focus of the processing industry is to maintain the organoleptic and nutritional characteristics and ensure the quality and safety of food products, the phytoextracts/phytochemicals derived from plants are gaining increasing importance nowadays. This paper aims to provide an overview of the recent applications of phytoextracts/phytochemicals for shelf-life extension and nutritional and organoleptic quality and sensory improvement of the fish, and its products.
-
Phytochemicals, also called green chemicals, produced by plants through primary or secondary metabolism, are gaining attraction as healthier alternatives to synthetic antioxidants and antimicrobials[14]. The phytochemicals are extracted from various plant parts (leaves, shrubs, seeds, flowers, fruits, bark etc.). They also can be recovered from the residues and leftovers of fruits and vegetables (peels, pulp) generated during harvesting and processing[15] and thus contribute to waste valorization and circular economy[11]. Generally, based on their chemical structure and characteristics, the secondary metabolic products of plants are classified into phenolics, terpenoids, carbohydrates, phytosterols, alkaloids and other nitrogen-containing compounds[11−13]. Phenolics are the largest category of phytochemicals being structurally diverse and abundantly distributed in the plant kingdom. They can be divided into phenolic acids (hydroxybenzoic acids − e.g. gallic, ellagic, vanillic acids etc.; hydroxycinnamic acids − e.g. caffeic, chlorogenic, cinnamic, ferulic etc.), flavonoids (flavones, flavonols, flavan-3-ols, isoflavones, anthocyanidins, anthocyanins etc.) and other phenolics (tannins, stilbenes, lignans, xanthones, lignins, chromones etc.)[16]. Among flavonoids, flavonols like quercetic, rutin, myricetin, kaempferol etc. and flavon-3-ols like catechin, epicatechin etc. are common. Apart from flavonols and flavones, anthocyanins and anthocyanidins are major flavonoids widely available in various fruits and vegetables such as grapes, apples, plums, cabbage, purple corn, and different varieties of berries like elderberry, blueberry, blackberry, elderberry, etc. Popular bioactive compounds among anthocyanins and anthocyanidins are cyanidin, malvidin, delphinidin, peonidin, petunidin, pelargonidin, etc.[11].
Terpenes, also known as terpenoids, exhibit diverse biological and pharmacological properties which are beneficial to humans. Based on the number of C5 isoprene unit, terpenes are grouped as hemiterpenes (prenol and isovaleric acid), monoterpenes (geraniol and limonene), sesquiterpenes (farnesol), diterpenes (quinogolides and taxadiene), sesterterpenes, triterpenes (squalene), tetraterpenes and ployterpenes[17]. Carotenoids such as lycopene, phytoene, phytofluene, lutein, zeaxanthin, β-cryptoxanthin, astaxanthin etc. are tetraterpenes reported to have many biological functions. These terpenoids form the major constituents of various essential oils.
Essential oils (EOs) obtained from various parts of plants (leaves, barks, stems, roots, flowers, and fruits) are complex mixtures of numerous individual aromatic volatile compounds that can act as defense mechanisms against microorganisms[18]. These volatile compounds belong to various chemical classes: alcohols, ethers or oxides, aldehydes, ketones, esters, amines, amides, phenols, heterocycles, and mainly the terpenes. Thousands of compounds belonging to the family of terpenes have so far been characterized and identified in essential oils[19], such as functionalized derivatives of alcohols (α-bisabolol), ketones (menthone, p-vetivone) aldehydes (citronellal, sinensal), esters (γ-tepinyl acetate, cedryl acetate), and phenols (thymol). Interestingly, EOs also contain non-terpenic compounds which are bio-generated by the phenylpropanoids pathway, like eugenol, cinnamaldehyde, and safrole[20]. Figure 1 illustrates the different kinds of natural bioactive compounds extracted from various plant components.
-
After harvest, different activities namely oxidative and enzymatic autolysis in fish cause deteriorative changes in sensory and nutritional value. These changes not only cause the loss of freshness as perceived by consumers but also limit the shelf-life during storage. Again above deteriorative changes with loss of freshness are mainly due to lipid oxidative products and protein degradation, which accelerate the undesirable changes in color, flavor and texture in fish. A wide range of plant extracts rich in polyphenols have been used for the preservation of fish and fish products, because of their antioxidant and antimicrobial activities. These extracts are applied either as dip treatment or as a coating in raw, chilled, and frozen stored products[21]. Various packaging methods viz. vacuum packaging and modified atmospheric packaging (MAP) are employed for extending the shelf life during the storage and retailing of fish and fish products. Different plant extracts are also being incorporated into ice to enhance the quality attributes of fish during storage[22]. In a recent study, the application of oregano essential oil (OEO) vapors under vacuum conditions, immediately before packaging, has been reported to be more effective than conventional dipping and topical application in maintaining the freshness and quality of fish products[23]. The effect of various phytochemicals on the quality aspects of fish and fish products is depicted in a schematic diagram shown in Fig. 2.
Figure 2.
Schematic diagram showing the effect of various phytochemicals on quality aspects of fish and fish products.
Physicochemical properties
-
Physicochemical properties like pH, water holding capacity, emulsion stability, cooking yield, etc., play important roles in case of emulsion-based muscle foods, including fish products. Amongst these, pH and water holding capacity are regarded as critical quality parameters in muscle food products due to their closest adherence to texture, cooking loss, juiciness, tenderness, and microbial quality of the products. Several reports on phytoextracts and their effects on the pH, water holding capacity, yield, total volatile basic nitrogen (TVB-N), trimethylamine (TMA-N) etc. of fish and fish-based products are available in the literature (Table 1).
Table 1. Effect of phytochemicals as bioactive compounds on physicochemical, microbiological and sensory quality of fish and fish products.
Fish and fish products Phytochemical used Parameters studied Key findings References Salted sardines
(Sardina pilchardus)Lemon essential oil (EO) micro-emulsion at 3 and 10 g/kg Chemical, microbiological and sensory parameters of salted sardines during the entire period of ripening (150 d) • Retarded the growth of Enterobacteriaceae by 0.95 CFU/g, Staphylococci by 0.59 CFU/g, and rod lactic acid bacteria by 1.5 log cycles
• Lowered the accumulation of histamine
• Registered highest scores for flavor and overall acceptability[46] Common carp (Cyprinus carpio) fillets Cinnamon essential oil (1 g/kg) Physicochemical, spoilage microbes and sensory attributes of fillets stored at 4 ± 1 °C for 14 d • Decreased the relative abundance of Macrococcus (51.8% vs 33.4%)
• Effective in inhibiting the increase of TVB-N and the accumulation of biogenic amines.
• Extended the shelf life of vacuum-packed fillets[40] Fillets of Sardinella longiceps and Rastrelliger kanagurta Coleus aromaticus and Sargassum wightii leaf paste Proximate, microbiological, and sensory characters of fillets under chilled storage (4 ± 1ºC) conditions for 7 d • Significantly (P ˂ 0.05) improved proximate parameters with reduced moisture and TVC content as compared to control
• Treated fillets had the best appearance, smell, color, texture, and taste compared to control
• S. wightii proved to be a better preservative than C. aromaticus[27] Smoked tilapia
(Oreochromis niloticus) fishGinger, garlic and clove powder
(5 g/kg)Microbial activity, shelf life and safety of fish during 8 weeks of storage • Preservatives treated samples had reduced microbial load, and longer shelf-life (8 weeks)
• Treated samples recorded zero/no total coliform count (TCC) growth and were accepted by the consumers[47] Frozen rainbow trout fillets Clove oil (5 and 10 g/kg) used as natural preservative Microbiological and sensory quality of frozen and vacuum-packed rainbow trout fillets stored at -18 °C for six months • Microbial growth was high for frozen storage for control samples
• Samples with clove oil had longer shelf life than normal
• Clove oil can be used as a natural protective and influential antibacterial in conjunction with a vacuum pack to augment the quality[64] Founder (Paralichthys orbignyanus) fillets Fillets packed in agar film with fish protein hydrolysate
(FPH) (500g FPH/ kg agar) or with film containing clove EO (500 g EO/kg agar)Microbiological quality and shelf life of fillets stored at 5 °C for 15 d • Fillets packaged with film containing clove EO had better microbiological quality than packaged in agar film with FPH
• Both the films effective in extending the shelf-life of fillets[65] Fish surimi from
O. niloticusTreating of surimi gel by immersion with colored plant extracts- CPEs
(2.5 g/L)- Hibiscus sabdariffa calyces, Curcuma longa rhizomes, and Rhus coriaria fruitsMicrobiological and sensory attributes of samples aseptically packaged into polyethylene bags and stored at 4 °C for 7 d • H. sabdariffa extract was the most effective antimicrobial
• CPEs enhanced sensorial attributes of surimi during storage study
• CPEs application as colorants and antibacterial and quality enhancing agents recommended for biopreservation of seafood[54] Fish patties from Hake fillets (Merluccius capensis, Merluccius paradoxus) Extract from pomegranate peel, rosemary, citric, and hydroxytyrosol (obtained from vegetable waters of olive tree)
(@ 0.2 g/kg)Physicochemical, microbiological properties, sensory analysis, and shelf life of patties under chilled storage for 14 d • Patties with rosemary extract had a high level of protein (140 g/kg),
α-linolenic acid (up to 400 g/kg), selenium minerals, and low fat
(< 20 g/kg) compared to the control
• Extracts effective against protein oxidation of patties than commercial preservatives added to the control
• Patties with pomegranate extract had a longer life (7 to 11 d) than others (4–6 d)[28] Grass carp (Ctenopharyngodon idellus) fillets Treatment with essential oils (EOs)- oregano (Origanum vulgare), thyme (Thymus mongolicus Ronn.), and star anise (Illicium verum) @ 1 g/L for 30 min at room temperature Microbial composition and quality of fillets stored at 4 ± 1 °C • EOs effective in inhibiting microbial growth (TVC) by 0.59 log CFU/g, delaying lipid oxidation, and retarding the increase of TVB-N, putrescine, hypoxanthine, and K-value
• Samples with EOs had a less fishy smell and firmer texture compared to the control
• EOs extended the shelf-life of fillets by 2 more days compared to the control
• Treatment with EOs can effectively inhibit the degradation of ATP and maintain a high quality of fish products[4] Sea bream (Sparus aurata) fresh fillets Application of oregano essential oil (OEO) in the vapor phase (67 µL/L) under vacuum (5–10 hPa) immediately before MAP fillet packaging Microbial and sensory product quality of fish fillets stored at 4 ºC for 28 d • OEO vapor treated samples had better physicochemical parameters (pH, TMA-N and WHC) as well as freshness compared to dipping
• Shelf life of vapor OEO treated MAP fish fillets was extended up to 28 d compared to control (7 d)
• Microbial quality of fish fillets is well preserved with the innovative OEO vapor injection under vacuum[23] Sardine (Sardinella albella) muscle Betel leaf (Piper betle) extracts (BLE)
@ 0.5 and 1 g/kg in the ice mediumMicrobial, biochemical, and sensory score of fish during 14 d of chilled storage • BLE at both concentrations inhibited the microbial proliferation and fish deterioration and extended the shelf life of fish for at least 3 d compared to the control sample
• BLE incorporated into ice improved the sensory score and chemical (pH, TVB-N, and TMA-N) quality[30] Indian mackerel (R. kanagurta) Methanolic extract of the red alga Gracilaria verrucose-GC @ (0.67 and 2.5 g lyophilized alga/L aqueous solution) in the icing medium Microbial, chemical, and sensory study of fish chill stored for 15 days • GC significantly (P < 0.05) inhibited the mesophilic and psychrophilic bacteria and chemical markers (pH, TVB-N, TMA-N, and biogenic amines) of fish deterioration relative to the control
• Icing medium containing GC extract improved the sensory acceptability, quality, and safety of fish compared to control
• The seafood industry can explore icing medium containing GC as a biopreservative[66] Hairtail fish ball Aqueous solution containing 1 g/kg sage extract, 1 g/kg oregano extract and
0.1 g/L grape seed extract (GSE)Quality and volatile flavor component of
fish balls stored at 4 °C up to 15 d• GSE stabilized meatball pH of hairtail fish
• The extract also reduced fishy odor, TBARS values, and TVB-N
• Inhibited bacterial growth compared to control[67] Wallago attu fish nuggets Treated with guava (Psidium guajava L.), bael (Aegle marmelos L.) pulp and dragon fruit (Hylocereus undatus L.) peel powder @ 15 g/kg Various physicochemical,
textural and sensory attributes of fish nuggets refrigerated stored up to 10 d• Fruits powder @ 15 g/kg significantly reduced the pH of the nuggets compared to control
• Increased emulsion stability, cooking yield, moisture, fat, and protein percentage
• Slowed down the lipid peroxidation of fish nuggets
• Textural attributes were improved in treated nuggets[25] Minced meat of Indian mackerel (R. kanagurta) Pomegranate peel extract (PPE)
@ 1, 1.5, and 2 g/kgOxidative stability of samples packed in polythene bags and stored at 4 °C • PEE @ 2 g/kg ppm increased oxidative stability of minced meat
• Improved shelf life of fish meat up to 8 days compared to 4 d in control[41] Canned common barbel (Barbus barbus) fish burgers Cystoseira compressa and Jania adhaerens powder @ 5, 10, and
15 g/kgTexture and sensory characteristics of fish burgers stored at 4 ºC for further analyses (8 months) • Treated formulations had improved nutritional content WHC, and enhanced texture stability
• Burgers containing 10 g/kg algae had better texture and sensory properties (P < 0.05)
• Algae could be considered as nutritious additives and natural flavoring and coloring agents to produce fish-based products[68] Cobia (Rachycentron canadum) fillets Psidium guajava extract (PGE)
@ 0.3 g/kg (w/v) for 30 minPhysicochemical and microbiological changes in fillets packed and stored in ice for 15 d • PGE @ 0.3 g/kg showed a significantly lower increment of pH values during storage
• Treated fillets showed significantly higher sensory properties, lower PV and TBARs compared to the control[24] Bighead carp (Aristichthys nobilis) fillets Aqueous pomegranate peel extract (APPE) @ 0.5 g GAE/L and ethanolic pomegranate peel extract (EPPE)
@ 0.5 g GAE/LMicrobiological and quality changes in fillets stored at 4 °C for 8 d • PPE decreased the TVC of fish spoilage bacteria such as Pseudomonas, Aeromonas, and Shewanella
• APPE is more effective in retarding the
increase of TVB-N and K-value
• EPPE was relatively better in inhibiting biogenic amines[52] Atlantic mackerel (Scomber scombrus) fillets Fillets immersed in 10 g/L of rosemary or basil essential oils (EOs) for 30 min at 2 °C Physicochemical quality of fillets stored at 2 °C up to 15 d • Rosemary and basil treatments effectively inhibit the formation of TVB-N and lipid oxidation products during storage.
• Significantly lower pH values were observed for the basil group than others, indicating antimicrobial effects
• Compared to the control group, fillets treated with rosemary and basil EOs had extended shelf life by 2 and 5 d[69] Common Carp (C. carpio) fillets Edible coating (C + EC), edible coating +, 5 g/kg chitosan (C + ECCh) and edible coating + 15 g/kg chitosan + 100 g/kg peppermint (C + ECChP) Quality and shelf life of common carp (C. carpio) during refrigerated storage (4 ± 1 °C) for 9 d • ECChP coating treatment extended the shelf life of carp by about 4 days compared with the control
• (C + ECCh) and (C + ECChP) significantly effective (P < 0.05) in delaying hydroperoxide production of fillets during refrigerated storage, reducing lipid oxidation[36] Fish (S. scombrus) mince Green tea
extract (GTE), grape seed extract (GSE), and pomegranate rind extract (PRE) at a level of 0.1 g/kg equivalent phenolicsChanges in quality of fish mince during frozen storage at −18 ± 1 °C for 6 months • PRE effectively inhibited lipid oxidation with lower peroxide and TBARS values
• Minced fish containing PRE had lower carbonyl and higher sulfhydryl contents
• GTE was not effective against lipid and protein oxidation
• PRE could be utilized as an antioxidant to extend the storage period in raw minced fish tissue[34] Fried fillets of Nile tilapia (O. niloticus) Fillets treated with rosemary extract-RE (1, 2, 3 g/kg) and Vitamin E 1 g/kg Physicochemical and sensory quality of fried fillets stored for 15 d at
4 ± 1 °C and 3 months at −18 ± 2 °C• TMA-N and TVB-N, values of RE and vitamin E treated samples were significantly lower than control samples (P < 0.05)
• R.E. @ 3 g/kg retarded oxidative changes in chilling and frozen fried fillets
• Significant (P < 0.05) enhancement in sensory quality attributes in samples treated with RE and vitamin E[59] Whole rainbow trout (Oncorhynchus mykiss) Effect of ice coverage comprised of Reshgak (Ducrosia anethifolia) extract (RE) @ 3 mg/L and Reshgak essential oil (REO) @ 15 g/L Chemical, microbiological and shelf life study during a 20-day storage period. • Treated samples had lower bacterial counts and chemical indices than ice coverage without extract
• Fish stored in ice containing REO had a longer shelf-life (> 16 d) than RE (16 d) and lot stored in traditional ice (12 d)[70] TMA-N = Trimethylamine; TVB-N = Total volatile basic nitrogen; TVC = Total viable count; TBARS = Thiobarbituric acid reactive substances; WHC = Water holding capacity; MAP = Modified atmosphere packaging. The cobia (Rachycentron canadum) fish fillets treated with guava leaf extract had significantly lower pH values than the control samples during storage for up to 15 d[24]. Likewise, the incorporation of different fruit powders viz. guava and bael pulp at 15 g/kg were found to significantly reduce the pH of fish nuggets[25]. The application of oregano EO in the vapor phase (67 μL/L) under vacuum (5−10 hPa) immediately before MAP of sea bream (Sparus aurata) fresh fillets has also been reported to maintain the physicochemical parameters like pH, TMA-N, water holding capacity and freshness[23]. The lower pH, TMA-N and freshness in treated fillets might be due to inhibition of spoilage microorganisms by EO vapors and reduced accumulation of alkaline compounds from protein degradation and decarboxylation of amino acids. Furthermore, the acidic nature and ascorbic acid content of plant extracts applied in powder form contributed to lowering the pH of fish products.
Sardine (Sardinella aurita) fillets marinated with pomegranate (Punica granatum L.) peel and artichoke (Cynara cardunculus L.) leaves extracts had the lowest pH value, TVB-N, and histamine content at the end of the storage time, resulting in less microbial spoilage compared to the control group[26]. In another study, the Coleus aromaticus leaf and microalga (Sargassum wightii) paste significantly improved protein, lipid, and carbohydrate contents while reducing moisture content of fish fillets of Sardinella longiceps and Rastrelliger kanagurta stored under chilled conditions for 7 d compared to control[27]. Apart from exhibiting antioxidative and antimicrobial activities, phytoextracts also preserved/improved the nutritional value of products. For example, fish patties incorporated with rosemary extract at 0.2 g/kg were found to have higher protein (140 g/kg), phosphorus and selenium minerals, alpha-linoleic acids up to 400 g/kg and low-fat contents (< 20 g/kg), compared to the control sample[28].
Fish nuggets with dragon fruit peel powder (10, 15 and 20 g/kg) have been reported with lower pH values, and significantly improved emulsion stability and cooking yield compared to the control[29]. This might be due to the water and fat-binding properties of dietary fibre present in dragon fruit peel powder. Betel leaf (Piper betle) extracts (0.5 and 1 g/kg) in ice medium have also been reported to improve the chemical quality such as pH, TVB-N, TMA-N and extend the shelf life of sardine muscle[30]. Essential oils like oregano, thyme, and star anise (1 g/L) were reported to be effective in delaying lipid oxidation, biogenic amines formation and TVB-N putrescine, and hypoxanthine of grass carp fillets. Besides, these EOs also delay the degradation of ATP and IMP which in turn helps in maintaining the quality of fish products[4].
Antioxidant activity
-
Fish oils contain unsaturated fatty acids, especially polyunsaturated fatty acids (PUFA) which are easily susceptible to oxidative changes. Hence fish and fish products are preserved for longer periods of time with additives having antioxidant properties. During the storage of food products, peroxide value (PV) and thiobarbituric acid (TBA) are considered useful indicators to determine the degree of lipid oxidation. In a study conducted by Mazandrani et al.[31], the peroxide and TBA values of silver carp fillets treated with liposomal encapsulated fennel extracts were significantly lower than the control during storage, suggesting that the fennel extract after encapsulation in liposome may be more effective in lowering lipid oxidation. The preservative effect of dried red beetroot peel (DRBP) extract was studied to monitor the quality changes, such as TBA and sensory values in Nile tilapia fish fillet. The fillets treated with DRBP extract at 1 g/L had reduced TBA content and acceptable sensory scores compared to non-treated samples[32]. The antioxidant potential of the peel extract primarily could be due to the presence of betaines, phenolic and flavonoid compounds containing amino and hydroxyl groups, and other active components such as carotenoids and glycine. In another study, aqueous extracts of seaweed (Padina tetrastromatica) applied at 2% as an additive has been reported to reduce the meat discoloration and fat oxidation in Pangasius fish fillets, and thus extending their storage life[33]. This could be due to tannic acids in seaweed extracts which reduced the myoglobin oxidation resulting in higher redness values. Pomegranate rind extract (PRE) at 0.1 g/kg equivalent phenolics was also reported to significantly reduce lipid oxidation (with lower peroxide and thiobarbituric acid reactive substances, TABRS) and protein oxidation (with lower carbonyl and higher sulfhydryl contents of fish mince during frozen storage)[34]. Protein oxidation can be assessed continuously by analyzing the carbonyl and sulfhydryl contents to understand the extent of protein damage during storage[35].
Edible coating of chitosan and peppermint has been reported to extend the shelf life of common carp (Cyprinus carpio) fillets during refrigerated storage, by delaying the hydroperoxide production, and also by reducing lipid oxidation[36]. The reduction of lipid oxidative products or delaying hydroperoxide production could be due to antioxidants present in chitosan and peppermint coating by quenching fatty acids or hydroxy radicals. In another study, basil leaf extract (10 g/L) was effective in inhibiting the formation of TVB-N and other lipid oxidative products in fillets of Atlantic mackerel (Scomber scombrus) during storage. Again, basil leaf essential oil combined with ZnO nanoparticles significantly lowered the production of TVB-N, biogenic amines, peroxide, and TBARS values during storage of sea bass (Lates calcarifer) slices[37]. The lower production of TVB-N in treated sample could either be due to the rapid inhibitory effect of bacterial growth or decreased bacterial capacity for oxidative de-amination of non-protein nitrogenous compounds, or both especially by basil leaf EO-ZnO nanoparticles film. Recently, hemp essential oil reinforced in nanoparticles with whey and mung bean proteins complex has been reported to inhibit the microbial activity, lipid oxidation and TVB-N in rainbow trout fillets during refrigerated storage[38]. Nisin and EO from Mentha pulegium. L. when used in free and nanoliposome forms minimized the growth of spoilage microorganisms, TVB-N production and improved sensory properties of minced fish[39]. In the aforesaid studies, amino acid decarboxylase inhibiting properties of EOs might be the reason for the low levels of TVB-N and biogenic amines in treated fish products during storage[40]. Pal et al.[41] reported the presence of high content of phenolic compounds like punicalagin, punicalin, gallic acid, and ellagic acid in pomegranate peel extract (PPE) which extended the shelf life of Indian mackerel mince (by up to 8 d). This could be due to higher antioxidant activity of ethanolic extract of pomegranate peel, when used at a concentration of 2 g/kg as compared to control.
Betel leaf extract in ice medium has been reported to significantly extend the shelf life of sardine muscle by reducing the production of TVB-N and TMA-N during storage[30]. Recently, extracts from various fruits such as blueberry, acerola, and grape have significantly inhibited the formation of heterocyclic aromatic amines (HAAs) in roasted yellow croaker. Particularly, blueberry extract was more effective in reducing the Norharman (94.85%) and heterocyclic amine 2-amino-1-methyl-6-phenylimidazo[4-5-b]pyridine (PhIP) (71.15%) content compared to fruit extracts[42]. Different fruits extracts possibly hindered the pyridines and pyrazines via Strecker degradation, derived from various precursors, including amino acids and glucose, responsible for formation of HAAs[43]. Interestingly, the use of herbs such as parsley (40 g/kg), chives (40 g/kg), and their mixture (Brazilian cheiro-verde) effectively inhibited the formation of cholesterol oxidation products (COPs) in grilled sardine fish[44]. As the formation of higher PhIP content in cooked muscle foods is considered mutagenic and carcinogenic, natural extracts from plant components could be an effective approach to minimize the formation of HAAs in muscle food products.
Antimicrobial activity
-
Available reports suggest that essential oils from plants, when applied as natural preservatives, show good antimicrobial activity, and maintain the quality of fish and fish products during storage. Antimicrobial effect of EOs is mainly due to interaction of hydrophobic part oil with lipid components of cell membrane of the bacteria resulting in change in sequences of metabolic function and cell death[45]. The addition of lemon EO micro-emulsions retarded the growth of Enterobacteriaceae, Staphylococci, and rod-shaped lactic acid bacteria in salted sardines[46]. Likewise, hot smoked tilapia (Oreochromis niloticus) treated using EOs (extracts of ginger, garlic, clove) had significantly lower total viable, total psychotropic, lactic acid bacteria count for 7 weeks storage period[47]. The inhibitory effects of olive by-products (such as olive leaf extract-OL, olive cake-OC, and black water-BW) were studied on fish spoilage bacteria from anchovy, mackerel, and sardine. Kuley et al.[48] reported that OL extract was more sensitive to fish spoilage bacteria and reference strains such as Enterobacter cloacae, Serratia liquefaciens, Proteus mirabilis, Photobacterium damseale, Pseudomonas luteola, Pantoea spp., Vibrio vulnificus, Stenotrophomonas maltophila, Acinetobacter lwoffii, Pasteurella spp., and Citrobacter spp. The effect of salep gum containing orange peel essential oil (2.5 and 5 g/kg) coating on the microbial growth and shelf life of rainbow trout (O. mykiss) fish fillets stored for 16 d under refrigerated conditions was investigated[49]. Samples treated with 5g/kg orange essential oil had improved shelf life and low numbers of total aerobic mesophilic, psychrophilic, coliforms and lactic acid bacteria, which can be ascribed to the presence of antimicrobial compounds (limonene and other minor compounds) in orange essential oil, exhibiting antimicrobial effects.
The EO of Zataria multiflora Boiss (ZMB) was reported to be more sensitive, particularly to Gram-negative more than Gram-positive bacteria that cause seafood spoilage, hence can be used as a natural additive for food preservation[50]. However, Hosseini et al.[51] indicated that the highest concentration with sensory acceptability of ZMB EOs when used in rainbow trout cannot inhibit the growth of L. monocytogenes at room, and optimum growth temperature. The variation in antibacterial effect of EOs may be due to factors such as the type, concentration, and form of EOs (liquid or vapor), number of microorganisms and influence of food matrix such as low pH value, level of sodium chloride etc. The vapor phase of various EOs has also been reported to have antimicrobial activity in various food systems. In a recent study, the vapor phase of oregano EO under vacuum immediately before MAP packaging has been reported to limit the microbial growth and maintain the quality of sea bream fresh fillets during refrigerated storage for 28 d. Chemically, the vapor phase of EOs being hydrophobic in nature are accumulated in the lipid component of microbial cell membrane hampering its functional properties, thus leading to structural damage[23].
Various researchers have reported the antimicrobial effect of pomegranate peel extract (PPE) on the quality and shelf life of fish and fish products, mainly due to the presence of higher phenolic components such as punicalagin, gallic acid, ellagic acid, chlorogenic acid, caffeic acid, catechin, epicatechin, rutin, quercetin, and galangal[26]. Zhuang et al.[52] reported that bighead carp (Aristichthys nobilis) fillets treated with PPE at 0.5 g GAE/L had significantly decreased total volatile compounds (TVC) of fish spoilage bacteria such as Pseudomonas, Aeromonas, and Shewanella, during chilled storage. The efficacy of pomegranate peel powder breaded on ready-to-cook cod sticks was studied against total mesophilic, psychrotrophic and other spoiling bacteria, Pseudomonas spp., Shewanella putrefaciens and Photobacterium phosphoreum[53]. The study reported a delayed microbial growth in treated samples stored for 17 d under refrigerated conditions, which could be due to higher bioactive compounds such as polyphenols, tannins, flavonoids and anthocyanins in peel powder, exhibiting antibacterial activity. Even colorants obtained from plant extracts such as Hibiscus sabdariffa calyces, Curcuma longa rhizomes, and Rhus coriaria fruits are reported to exert an antimicrobial effect on standard microbial stains like Escherichia coli (ATCC 25922), Salmonella typhimurium (ATCC 14028), Staphylococcus aureus (ATCC 25923), and Pseudomonas aeruginosa (ATCC 27853) in surimi from O. niloticus[54]. Dip treatment of Deccan mahseer (Tor khudree) steaks with beetroot (Beta vulgaris) peel extract (200 g/L) has been reported to exhibit a positive effect by retarding spoilage, thereby extending shelf life up to six months during frozen storage study[55]. The addition of Simira ecuadorensis plant extract (80 g/kg) significantly reduced the aerobic mesophilic bacteria count in a fish burger[56]. The extract reduced the pH of fish burger thereby increased the microbiological safety during further storage periods. Betel leaf extract has also been reported to significantly lower microbial proliferation and prolongs the shelf life of sardine muscle for at least 3 more days compared to the control[30]. In a similar study, the fillets of O. niloticus treated with ethanolic extracts of betel leaf at 0.4 and 0.6 g/kg had reduced microbial growth and quality deterioration during 12 d of storage at refrigerated temperature, compared to untreated samples[57]. The minimum inhibitory concentration (MIC) of various phytochemicals against different fish spoilage microorganisms is presented in Table 2.
Table 2. Minimum inhibitory concentration (MIC) of phytochemicals against fish spoilage microorganisms.
Component of plants Fish products MIC values Target microorganisms References Curcuma longa rhizome powder (200 g/L of 70 % aqueous ethanolic solution) Surimi gel of tilapia 2.2 g/L
1.8 g/L
1.8 g/L
1.2 g/LSalmonella Typhimurium
Staphylococcus aureus
Escherichia coli
Pseudomonas aeruginosa[54] Gabsi pomegranate peel powder (5 g powder in
150 mL methanol for methanol pomegranate peel extracts)fresh fish 152 g/L E. coli
Saccharomyces cerevisiae[71] Olive leaf extract (OL), olive cake (OC), black
water (BW)Fresh anchovy, mackerel, sardine 3.0 g/L
6.0 g/L
12.5 g/LE. coli
Salmonella Paratyphi A
S. aureus[48] Betel leaf (Piper betle) powder (water extract) Sardine fish meat 0.5 g/L Psychrophilic bacterial count [30] Hibiscus sabdariffa
Calyces powder (200 g/L of 70% aqueous ethanolic solution)Surimi gel of tilapia 1.6 g/L
1.0 g/L
1.2 g/L
1.6 g/LS. Typhimurium
S. aureus
E. coli
P. aeruginosa[54] Thyme essential oil Minced fish meat 8 g/kg Listeria monocytogenes [72] Lavender essential oil Catfish 2 g/L,
1–1.2 g/LE. coli,
S. aureus[73] Kakadu plum bark powder (methanol extract) Chilled fish 1 g/L S. aureus [74] Fruits and culinary herbs of Australian plant powder (methanolic extract) Fresh fish 5 g/L Shewanella putrefaciens [75] Dried, fragmented leaves of rosemary, thyme and
dried fruits of anise (Pimpinella anisum)Canned fish 10 g/L (rosemary)
1.25 g/L (thyme)
10 g/L (anise)Clostridium perfringens [76] Simira ecuadorensis leaf powder (ethanol extract) Fish hamburger 80 g/L Campylobacter jejuni
and S. putrefaciens[56] Sensory evaluation
-
The use of phytochemicals in extract, powder, or oil forms influences the sensory attributes of fish and fish products at different levels of efficiency. Fish paste (pollack meat, cuttlefish meat, shrimp meat) received the best score in terms of taste and overall preference, when treated with different levels (10, 30, 50, and 70 g/kg) of C. longa powder[58]. Fish nuggets treated with dragon peel powder at 15g/kg had improved shelf life and sensory attributes compared to others during 15 d of storage[29]. Fillets of S. longiceps and R. kanagurta treated with leaf paste of C. aromaticus and S. wightii had the best appearance, smell, color, texture, and taste compared to control[27]. Even ethanolic extracts of betel leaf at 0.4 or 0.6 g/kg has been found to extend shelf life without any change in taste or discoloration of Nile tilapia (O. niloticus) fillets up to 9 d[57]. In a recent study, fried fillets of Nile tilapia (O. niloticus) treated with rosemary extract (1, 2, 3 g/kg) and vitamin E (1 g/kg) has shown significant enhancement in sensory characteristics[59]. Likewise, salted sardines (Sardina pilchardus), treated with lemon essential oil microemulsion received the highest scores for flavor and overall acceptability[46]. Berizi et al.[60] reported that a 10 g/kg concentration of methanolic pomegranate peel extract (MPPE) had the highest sensory rating and chewiness of chilled gutted rainbow trout. MPPE is, therefore, recommended as a natural agent to improve the textural properties of frozen fish during the first six months of storage. Panza et al.[61] adopted a zero- waste approach by utilizing the whole pomegranate (juice, peel, and seed) in varied proportions to find the effect on spoilage microorganisms and sensory quality of fish burgers. The researchers corroborated that pomegranate treated fish burgers had delayed microbial proliferation and maintained the sensory attributes with prolonged shelf life, due to the antibacterial action of tannins and phenolic acids present in the formulation.
As far as the impact on sensory acceptability, the effect of oregano EO at 12.5 g/L on texture, color, and sensory acceptability of balls prepared from Tambaqui (Colossoma macropomum) fish was evaluated. It was found that OEO improved the color and aroma as sensory attributes[62]. This could be due to the antimicrobial and antioxidative properties of EOs. The EOs inhibit the H2S-producing bacteria, and chemical reactions which are responsible for the development of off-odors. Besides, when treated with thyme and star anise essential oil (1 g/L) at room temperature for 30 min, grass carp fillets had a less fishy smell and firmer texture than the control[4]. Although the above concentrations (v/v) were acceptable by the panelists.
To overcome this, combination of various plant derived EOs is suggested, that possess high phenolic content but effective at low concentrations, so that their synergistic effect may offer better antimicrobial and antioxidant activities, and consequently maintaining the balance between sensory properties of fish products and odor factor of EOs. Encapsulation technique is also reported to be effective in masking the strong odor and flavor of EOs, as it (i) maintains the inherent flavor characteristics of food, (ii) prevents the evaporation of volatile compounds, (iii) enhances solubility for effective release and better distribution[11, 63]. It is recommended that materials used for encapsulation should have low reactivity with EOs, to ensure limited impact on the sensory attributes of foods.
-
Natural preservatives are safer and more effective agents for retarding the deterioration process of fish products. Because of this, plant-derived bioactive compounds and secondary metabolites, also called phytochemicals/green chemicals, with antioxidant and antimicrobial characteristics are now preferred over their synthetic counterparts. The application of phytochemicals as food additives extends the shelf life by delaying lipid oxidation and inhibiting microbial growth, thereby ensuring better nutritional value, and improved textural properties of fish and fish products. The increased demand for high quality fish products and the concept of 'Green consumerism' gaining momentum are boosting the application of bioactive phytochemicals, obtained from available, cheap, and underutilized resources. Modern and green extraction methods, including ultrasound-assisted extraction, microwave-assisted extraction, supercritical fluid extraction, and pressurized liquid extraction may be employed to obtain enhanced yields and stability of the phytochemicals. Further, the suitability of the phytochemicals, and their synergistic effect in combination with other natural preservatives or non-thermal technologies (irradiation, high pressure, retort pouch processing) and innovative packaging technologies may be explored to increase the degree of quality, functionality, sensory acceptability as well as shelf life of the fish and fish products, and thus meet consumer expectations.
-
Thanks to the Director, ICAR-Indian Veterinary Research Institute (IVRI), Izatnagar, Bareilly, India and the Station In-charge, Eastern Regional Station, ICAR-IVRI, Kolkata, India for their encouragement in writing this manuscript.
-
The authors declare that they have no conflict of interest.
- Copyright: © 2023 by the author(s). Published by Maximum Academic Press on behalf of Nanjing Agricultural University. This article is an open access article distributed under Creative Commons Attribution License (CC BY 4.0), visit https://creativecommons.org/licenses/by/4.0/.
-
About this article
Cite this article
Biswas O, Kandasamy P, Nanda PK, Biswas S, Lorenzo JM, et al. 2023. Phytochemicals as natural additives for quality preservation and improvement of muscle foods: a focus on fish and fish products. Food Materials Research 3:5 doi: 10.48130/FMR-2023-0005
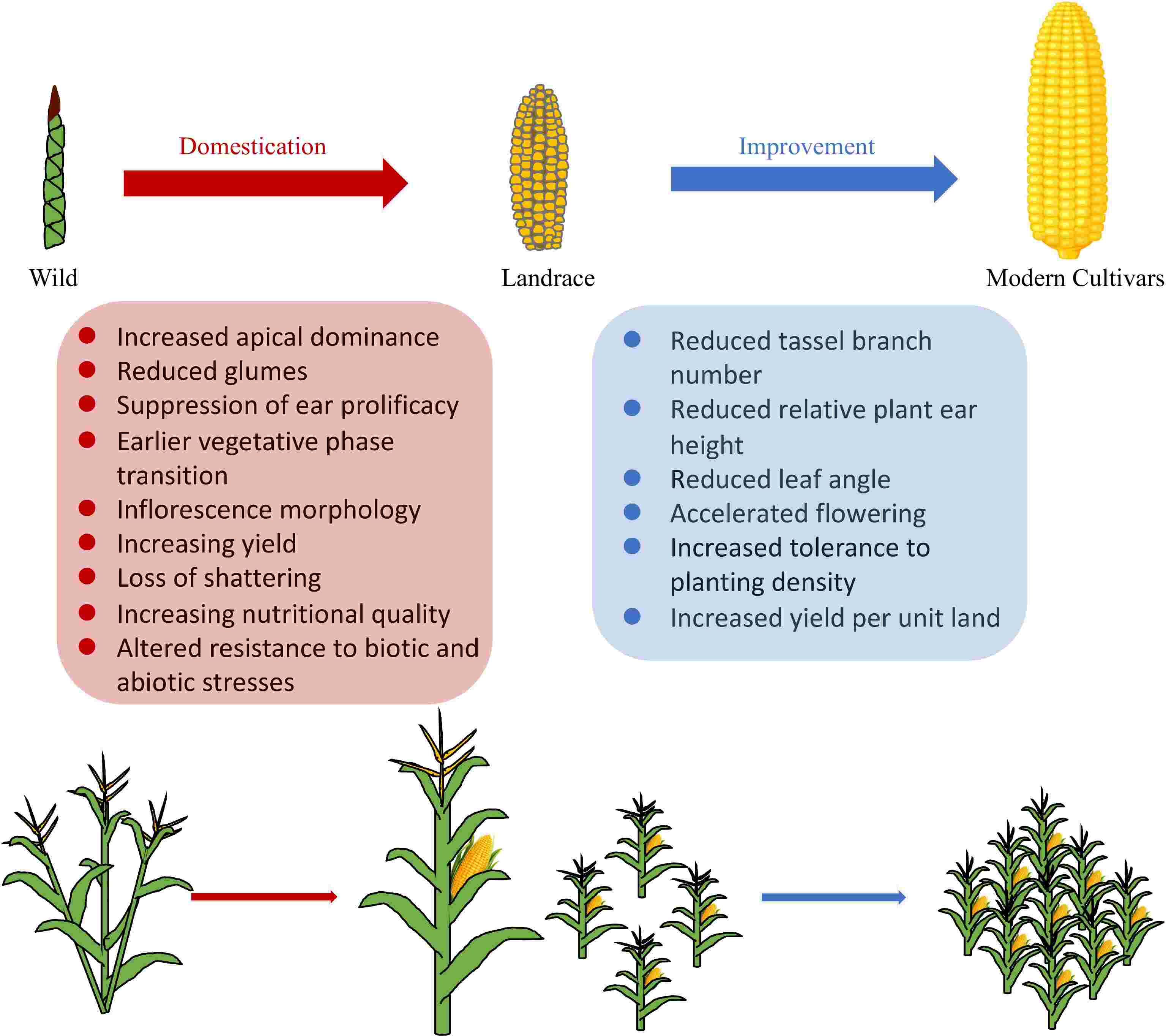