-
Areca nut, derived from the seeds of the Areca catechu L. palm, stands as a traditional commodity deeply rooted in the cultures of Asia, East Africa, and the Western Pacific[1]. Chewing areca nut is an ancient custom followed by the people living in these areas to obtain relaxation, better concentration, and euphoria, and statistically, adult chewing rates range from 2.3% in China to 47.8% in Indonesia[2,3]. The constituents within the areca nut encompass diverse compounds, including polysaccharides, flavonoids, fatty acids, and alkaloids[4]. Among these components, alkaloids stand out as the primary active constituents, and arecoline constitutes a significant proportion of 0.3%−0.6%[1,5]. Approximately 600 million individuals worldwide consume areca nut, making arecoline the most commonly used substance by humans after alcohol, caffeine, and nicotine[2].
Historically, areca nut has served as a medicinal plant with ancient roots. Areca nut occupies an essential position in traditional Chinese medicine classics such as the Compendium of Materia Medica and is often used to treat gastrointestinal disorders such as dysentery, bloating, and constipation[1]. Modern studies have shown that arecoline, the main active ingredient in areca nut, stimulates intestinal smooth muscle contraction and promotes intestinal peristalsis by stimulating muscarinic acetylcholine receptor (mAchR) and voltage-gated potassium channels, thus improving intestinal health[6,7]. In addition, as a psychoactive substance, arecoline can alleviate spatial working memory deficits in neurodivergent mice and cognitive deficits in Alzheimer's patients under specific conditions, demonstrating therapeutic potential for neurological disorders[8,9].
However, in 2020, the International Agency for Research on Cancer classified arecoline as 'probably carcinogenic to humans' (Group 2B carcinogen) based on compelling mechanistic evidence[10]. Approximately half of oral cancers reported are attributed to areca nut chewing in the Indian subcontinent and Taiwan[11]. When chewing areca nut, the oral cells are rubbed by areca nut fibers and infiltrated by arecoline, prone to inflammatory reactions and collagen disorders, forming oral mucosal fibrosis, a type of oral precancerous lesion[12]. In addition, increased oxidative stress, epigenetic dysregulation, and immune dysfunction due to arecoline may also be an important cause of oral cancer[13−15]. Arecoline can affect virtually every organ in the body, including but not limited to neurotoxicity[16], cardiotoxicity[17], causing asthma[18], and decreasing embryonic viability[19]. Given the widespread use of arecoline, it is particularly urgent to clarify the pharmacologic and toxicologic effects and mechanisms of arecoline on various organs.
In this review, we briefly discuss the multifaceted actions of arecoline on various organs, considering pharmacological and toxicological perspectives, and offering a nuanced understanding of how arecoline affects different physiological systems. We delve into the health functions of arecoline on vital systems, including its influence on neurotransmitter modulation, smooth muscle contraction, and the notable antiparasitic properties of arecoline. We also underpin the toxic effects of arecoline on critical organ systems, encompassing factors like fibrosis, oxidative stress, immune dysfunction, and epigenetic alterations. Given the extensive discussions surrounding arecoline, we aim to advance the understanding of its intricate pharmacological and toxicological profiles, ultimately paving the way for developing therapeutic strategies.
-
Arecoline has been observed to accumulate in the oral cavity after entry, posing a significant risk to oral health. Salivary concentrations of arecoline in volunteers chewing 0.5 grams of areca nut ranged from 5.66 to 97.39 µg/mL[20]. Even after a brief chewing period, 100 ng/mL residual concentrations are common[21]. In vitro, arecoline can stimulate cultured cells at concentrations as low as 0.1 µg/mL and is cytotoxic at 10 µg/mL[20]. Epidemiological studies have established a correlation between regular consumption of areca nut and potentially malignant oral diseases, such as oral submucous fibrosis (OSF) and oral squamous cell carcinoma (OSCC) (Fig. 1).
Figure 1.
Possible mechanisms of oral submucous fibrosis (OSF) and oral oral squamous cell carcinoma (OSCC) induced by arecoline.
Oral submucous fibrosis (OSF)
-
OSF is a kind of oral potentially malignant disorder, mainly caused by areca nut chewing[22]. The physical friction of arecoline coarse fiber and the chemical irritation of arecoline can cause damage and inflammation to oral tissue, and long-term chewing habits can lead to abnormal and persistent tissue inflammation, which is a critical factor in developing cancer and tissue fibrosis[23]. Various inflammatory mediators play pivotal roles in these pathogenic processes. Arecoline is implicated in stimulating the cellular expression of pro-inflammatory and pro-fibrotic cytokines, including prostaglandin E2 (PGE2), interleukin-6 (IL-6), tumor necrosis factor α (TNF-α), transforming growth factor-β (TGF-β) and cyclooxygenase 2 (Cox-2)[23,24]. Among those inflammatory factors, arecoline enhances collagen synthesis[25], increases procollagenase levels[26], and upregulates lysyl oxidase activity (a key enzyme in collagen fiber processing)[27] by inducing TGF-β signaling[24]. Arecoline also induces the expression of connective tissue growth factor (CTGF), a downstream target of TGF-β, by activating mitogen-activated protein kinase (MAPK) and nuclear factor kappa-light-chain-enhancer of activated B cells (NF-κB)[28]. Additionally, in oral keratinocytes-fibroblasts, tissue inhibitor of metalloproteinases 1 (TMP1), an inhibitor of enzymes involved in extracellular matrix (ECM) degradation, exhibited increased production following arecoline pre-treatment[29].
Another possible OSF route is epithelial-mesenchymal transition (EMT). Zinc finger E-box binding homeobox 1, a transcription factor that instigates EMT, experiences increased expression under the influence of arecoline. This upregulation drives the expression of α-smooth muscle actin (α-SMA) via activation of the α-SMA promoter, thus prompting the differentiated metastasis of myofibroblasts in buccal mucosal fibroblasts, subsequently contributing to ECM accumulation and participating in OSF pathogenesis[30]. Moreover, Twist is another EMT transcription factor that plays a role in arecoline-associated OSF by regulating collagen contraction and wound healing capacity in OSF[31]. Overall, stimulation of the immune system, TMP1, and EMT, leading to disturbances in collagen homeostasis, are possible mechanisms by which arecoline causes OSF, as detailed in Fig. 1.
Oral squamous cell carcinoma (OSCC)
-
OSF is a precancerous lesion that may precede the OSCC diagnosis[32]. According to the Global Cancer Observatory, in 2020, there were 377,713 cases (2.0%) of lip and oral cancer and 177,757 deaths (1.8%)[33]. OSCC accounts for more than 90% of oral cancer cases, with a five-year survival rate of 40%−50%[34]. Approximately half of oral cancers reported are attributed to areca nut chewing in the Indian subcontinent and Taiwan[11]. In mouse models, a standard method for simulating oral tumors is a combined treatment with arecoline and 4-nitroquinoline-1-oxide[35]. These findings support that OSCC has a significant association with arecoline. Existing studies demonstrate that arecoline may cause OSCC by inducing increased oxidative stress, epigenetic dysregulation, and immune dysfunction.
Arecoline induced the production of reactive oxygen species (ROS) and reduced expression of antioxidant enzymes, leading to chromosomal damage and gene mutations[13], one of the pathogenic mechanisms of OSCC. Typically, the body's ROS is in equilibrium with the antioxidant system, but sometimes, this equilibrium can be disturbed, such as after arecoline ingestion. When 50−200 μg/mL arecoline was used to treat gingival epithelial Smulow-Glickman cells and oral epithelial cell lines OEC-M1 and SAS, it induced ROS production and inhibited catalase expression, caused DNA double-strand breaks and activated the DNA repair response, which was characterized by down-regulation of ATM mRNA expression and an increase in p-ATM and γ-H2AX expression in the cells[13,36]. The tumor suppressor gene p53 regulates almost all DNA repair pathways[37]. Tsai et al. found that arecoline can inhibit the p53-regulated p21WAF1 promoter and p53 protein expression in KB and HEp-2 cells, inducing cell cycle arrest in S and G2/M phases[38]. Apart from these changes, arecoline also increases the phosphorylation levels of Wee1 kinase and Cdc2Tyr15 associated with S and G2/M phase arrest in KB epithelial cells[39], as well as regulating the expression of cell cycle protein D1, cell cycle protein A, cell cycle protein E, CDK4 and CDK2 in HaCaT keratinocytes[40].
Arecoline also induces epigenetic changes in oral cells, including alterations in non-coding RNA and DNA methylation, which can lead to the development of OSCC. MicroRNA is an endogenous non-coding single-stranded RNA molecule[41]. In the ORL-48(T) squamous cell carcinoma cell line, 0.025 μg/mL arecoline decreased miR-22 expression in cells, leading to reduced inhibition of oncostatin M, an IL-6-family inflammatory cytokine, which then promotes OSCC proliferation[14]. Similarly, in OSCC cell lines, arecoline could also participate in OSCC proliferation and metastasis by inhibiting miR-886-3p[42]. In OSCC patients and mice, arecoline promotes OSCC proliferation by reducing the expression of miR-329 and miR-410 genes, inducing the expression of Wnt-7b and β-catenin proteins[43]. Abnormal DNA methylation is another common epigenetic change in OSCC. Under arecoline exposure, miR-30a and miR-379 levels were reduced in OSCC cells, targeting increased DNMT3B expression, which mediated the downregulation and methylation of ADHEF1 and ALH1A2 involved in retinoid metabolism to promote the progression of OSCC[44]. In addition, arecoline exposure resulted in hypomethylation of PTK6 with increased PTK6 expression, which increased the proliferation rate, migration, and invasion of OSCC cells, as demonstrated in mice[45].
As the body's defense barrier, the immune system is important in recognizing and rejecting tumors. As early as 2001, it was noted that areca nut/arecoline reduced IL-2, TNF-α, TGF-β, and interferon-gamma (IFN-γ) levels in mononuclear cells of normal subjects and patients with squamous cell carcinoma[15]. In OSCC cell lines, arecoline increased the production of the pro-inflammatory factor IL-β partly through inflammasome, and IL-β induced angiogenesis and EMT, thereby promoting OSCC invasiveness[46]. Likewise, arecoline stimulated the production of PGE2, inhibited the expression of CD69 on CD4+ and CD8+ T cells in cellular KB oral cancer cells[23]. Furthermore, arecoline also increased the expression of obesity-associated protein, which regulates the expression of programmed cell death-ligand 1 via m6A modification and myc, as a means to increase the resistance of OSCC cells to CD8 T cells, thereby conferring the ability to immune escape from OSCC cells[47]. Based on the results of the current studies, long-term use of arecoline may increase the risk of OSCC. Therefore, reducing or avoiding exposure to arecoline is one of the most critical steps to prevent the risk of OSCC. In conclusion, both in vivo and in vitro studies support that arecoline impairs oral health.
-
There is a proverb in Hunan Province, China—areca nut and smoke; mana is boundless; areca nut and wine, get everything you want; areca nut, smoke, and wine, live to 99[48]. As a fat-soluble tertiary amine, arecoline crosses the blood-brain barrier well to enter and modulate the CNS, delivering a wide range of bodily effects, including euphoria, cognitive modulation, and addiction (Table 1)[2].
Table 1. Effect of arecoline on the CNS.
Effect Animal/cell Specific effect Pathway/mediators Dose Ref. Beneficial effects Xenopus laevis oocytes Anti-inflammatory activity As a silent agonist of α7 nAChR, targeting and regulating intracellular signaling against inflammation and pain / [61] Glioblastoma cell lines (U373 and U87MG) Interfere with the aggressiveness of malignant gliomas Inhibition of intermediate conductance Ca2+-activated K+ channels 10 and 30 μM [62] Zebrafish Cluster disruption and increased social interaction Increased norepinephrine, serotonin, and DOPAC levels decreased 5-hydroxyindoleacetic acid/serotonin level, and homovanillic acid/dopamine ratios 10 mg/L [55] Zebrafish Motor hyperactivity Binds with multiple mAChRs (M1−M4) to induce hyperactivity 0.001, 0.01, 0.1,
and 1 ppm[49] Male Swiss albino mice Antinociception By activation of central muscarinic receptors 0.3−1 mg/kg ip [53] Rat Attenuated a time perception impairment induced by daily scheduled feeding By modulating central cholinergic 10 mg/kg/d [52] Rat Anti-phenobarbital sodium-induced sleep time Not mentioned 0.5 mg [51] Male ICR mice Shortened the duration of
ethanol-induced sleepActs as a muscarinic agonist to relieve ethanol-induced central depression and intoxication 0.125−1.0 mg/kg, s.c. [50] CPZ mice Attenuating memory impairment and demyelination Acts as a muscarinic receptor 1 cholinergic agonist to improve cognition and promote myelination processes in the frontal cortex 2.5 or 5 mg/kg/d [8] Female BALB/c mice Increased the activity of preactivated NK cells By stimulating the secretion of corticotropin-releasing hormone and adrenocorticotropic hormone 1.5 mg/kg [63] Male albino rats Improved retrieval and memory storage in the stair maze Not mentioned 0.5 mg/kg [64] Human (Alzheimer) Low-dose arecoline improved cognitive performance, highest-dose impaired psychomotor activation By modulating central cholinergic 1, 2, or 4 mg/h infusions 2 h [9] Human (Alzheimer) Improved memory As a cholinergic agonist, maintaining patients’ cholinergic steady-state 0.042−1.7 mg/h Infusion for 11−16 d [65] Human (Alzheimer) Improved cognition As a muscarinic receptor agonist, regulating patients’ cholinergic system 0.5, 1, 2, 4, 8, 16, 22,
28, 34, and 40 mg/d[66] Neurotoxicity Primary cortical neuron Induction of neuronal cell death By attenuating antioxidant defense and enhancing oxidative stress 50−200 μM [16] PC12 Cells Apoptosis By inducing endoplasmic reticulum stress, attenuating H2S levels, CBS and 3-MST protein expression 0.5−2 mM [58] Drosophila melanogaster Neurotoxic agent and affected the life cycle parameters By reducing acetylcholinesterase and MAO, increasing caspase-3, caspase-9 activity, and oxidative stress 20, 40 and 80 μM [67] Zebrafish Dyskinesia By increasing ROS, endoplasmic reticulum stress, apoptotic p53 signaling pathway. 10 μM [68] Male albino rats Decreased correct responses and accelerated spontaneous decay of memory Not mentioned 3.5 and 8 mg/kg [64] Addiction-related Xenopus laevis oocytes Habitual use By activating addiction-related nAChR activity, receptors containing α4, β2, α6 and β3 subunits / [61] Xenopus laevis oocytes Addiction By activating α4 nAChR 100 μM [62] Zebrafish Withdrawal syndrome-like responses Not mentioned 1 mg/L [55] Pregnant women Exceptional adverse birth outcome Not mentioned Not mentioned [69] Excitability and improvement of cognitive impairment
-
The effects of arecoline on the CNS are complex; at some doses, arecoline can cause excitability and enhance cognitive performance. In zebrafish (Danio rerio) larvae, arecoline increases locomotor activity even at concentrations as low as 0.001 ppm[49]. In mice, arecoline shortens ethanol-induced sleep time (0.125 to 1.0 mg/kg)[50]. Arecoline also increases anti-1.5 × 10−4 phenobarbital sodium-induced sleep time by up to 38 min (0.5 mg)[51]. These phenotypes suggest that arecoline has significant excitatory effects. In addition, spatial memory impairment and brain demyelination were well alleviated in schizophrenic mice treated with 5 mg/kg/d arecoline[8]. Daily arecoline injections of 10 mg/kg attenuated the impairment of mealtime-associated activity on the fasting day in old rats[52]. In Chinese medicine, herbs with areca nut as the main ingredient can manage palpitations, insomnia, and mental irregularities[1]. In clinical practice, a low dose of arecoline can improve cognitive impairment, emotional capacity, and psychomotor activity in Alzheimer's patients[9]. It was found that arecoline is an agonist of mAChRs, which may promote body excitability and antinociception effects and improve learning and memory by activating the M1 muscarinic receptor subtype[53,54]. Additionally, arecoline exposure increased dopamine levels in the brains of mice and zebrafish, which may also be a reason for arecoline's ability to promote excitation in the organism[55,56]. These findings indicate that arecoline enhances cognitive performance and induces organic excitability, possibly by modulating neurotransmitter homeostasis in the brain.
Neurotoxic effects
-
Surprisingly, as the concentration increases, arecoline begins to disrupt the oxidative and antioxidant balance in the body, inducing neurotoxicity and apoptosis. NADPH oxidase (NOX) is a key enzyme for redox signaling and a significant source of ROS cluster in vivo[57]. Cellular experiments indicated that arecoline at concentrations of 50−200 μM can increase ROS by upregulating NOX2 levels and decrease glutathione (GSH) and superoxide dismutase (SOD) levels, causing redox imbalance in neurons. In this state, the expression of pro-apoptotic proteins (cytochrome c, Bax, caspase-9, and caspase-3) was increased, and the manifestation of anti-apoptotic protein Bcl-2 was diminished, which finally induced neuronal cell death[16]. Jiang et al. suggested that arecoline can induce neurotoxicity by causing endoplasmic reticulum stress in neuronal cells and interfering with endogenous H2S synthesis[58]. Moreover, zebrafish showed elevated expression of the protooncogenes c-fos and c-jun in the brain after 10 mg/L arecoline treatment, which was associated with cancer transformation and progression[55].
Addictive effect
-
After long-term consumption of areca nut, users may experience dependence such as tolerance, loss of control, craving, and salience, with surveys indicating that a high percentage of current users are dependent, accounting for 40% to 80% of the total[59]. Once discontinued, users may experience withdrawal symptoms, including mood swings, anxiety, irritability, and insomnia[2]. Dependency mechanisms are usually associated with the brain's dopamine system. As early as the 1980s, researchers showed that arecoline increased dopamine levels in the mouse cortex[56], and this was validated by the results that zebrafish exposed to acute arecoline increased brain levels of norepinephrine, serotonin, and the dopamine metabolite 3,4-Dihydroxyphenylacetic acid (DOPAC)[55]. Chen et al. emphasized that this increased dopamine may be partially derived from Monoamine oxidase A (MAO-A) inhibition, and MAO-A activity was indeed inhibited in neuroblastoma SH-SY5Y cells and rats after arecoline treatment, and individuals carrying the at-risk MAO-A allele were more likely to exhibit a dependent response in the population survey[60]. Furthermore, the α4β2 nicotinic acetylcholine receptor (nAChR) in the brain is a crucial regulator of the limbic dopamine system in the midbrain, and arecoline may mediate the rewarding effects behind habitual arecoline use through activation of the α4β2 nAChR[61].
The studies above have shown that the effects of arecoline on the CNS are complex. At lower doses, arecoline stimulates acetylcholine receptors, improving cognition and euphoria. However, higher doses of arecoline can induce neurotoxicity, apoptosis, and cancer transformation in the CNS. Prolonged intake and abuse of arecoline can lead to addiction, tolerance, and dependence through the release of dopamine in the brain. The positive cognitive enhancement effects of arecoline can be utilized to provide a new therapeutic idea for related diseases. However, it is important to be aware of the potential neurological problems that may arise from long-term use and abuse of arecoline to safeguard our neurological health.
-
The China Cardiovascular Health and Disease Report 2021 projects that cardiovascular disease now affects 330 million people and is currently the leading cause of death for the population, accounting for more than 40%[70]. Areca nut chewing may increase the risk of cardiovascular diseases[71], for instance, following areca nut chewing, one patient with coronary artery disease experienced an acute myocardial infarction[72], while two other patients experienced ST-elevation myocardial infarction[73]. Tseng et al. pointed out that when arecoline concentration was higher than 0.2 mM, it caused contraction, rounding, and shedding of EAHY cells, weakened the wound closure activity of EAHY cells, and promoted the adhesion of monocytes to EAHY cells, which is an early step in atherosclerosis[74]. Furthermore, arecoline causes atherosclerosis by interfering with low-density lipoprotein (LDL) receptors to inhibit endocytosis of LDL cholesterol and interfering with high-density lipoprotein (HDL) receptors to prevent hepatic uptake of HDL cholesterol[71].
In addition to atherosclerosis, Goto et al. found that a subfraction isolated from areca nut (concentration above 3 × 10−7 μg/mL) had vasodilatory effects and relaxed rat aorta with intact endothelium-containing[75]. In anesthetized dogs, only 10 ng/kg of arecoline was able to reduce arterial blood pressure. When the dose was increased to 30 or 100 μg/kg, the experimental animals even showed a sustained interruption of cardiac activity[76]. Mice also showed a decrease in blood pressure after arecoline injection[77], suggesting arecoline may have similar, evolutionarily conserved cardiovascular system effects in animals such as humans, canines, and mice.
Cardiotoxicity
-
Studies have established an association between arecoline and cardiovascular disease, but the underlying mechanisms regarding arecoline-induced cardiotoxicity are poorly understood. Similar to the induction of oral cell fibrosis, arecoline induces cardiomyocyte fibrosis by disrupting the balance of the extracellular matrix by affecting immunity (TGF-β) and the enzymes that synthesize and degrade the extracellular matrix. Specifically, when mice were fed arecoline above 5 mg/kg/d, significant fibrosis occurred in the heart; on the one hand, arecoline was able to increase the expression of TGF-β1 and its downstream molecule p-Smad2/3, CTGF and its transcription factor SP1 were in turn regulated by the p-Smad2/3 pathway to increase their expression, thus participating in cardiac fibrosis; on the other hand, arecoline increased plasminogen activator and plasminogen activator expression, which in turn is involved in cardiac fibrosis by inducing the expression of matrix metalloproteinases-9[78]. In a study by Lin et al., arecoline at 5 mg/kg/d and above-induced protein expression of Fas-ligand receptors (Fas) ligand, Fas and Fas-associated protein with death domain in Sprague-Dawley rat cardiomyocytes, followed by activation of caspase 8 and caspase 3, causing apoptosis[17]. When the concentration was increased to 50 mg/kg/d, the authors found that the expression levels of apoptotic factors (tBid, Bak, cytochrome c) were increased and survival biomarkers (Bcl2, BclxL) were decreased, and the mitochondrial apoptotic pathway was activated in rat cardiomyocytes[17]. In addition, recent experiments have shown that arecoline-treated mice develop signs of cardiac hypertrophy, which are associated with expression of the MEK5/ERK5 and JAK2/STAT3 signaling pathways, the MAPK signaling cascade, as well as calcium-regulated neurophosphatase and NFATc3[79].
Together, these findings support that arecoline may influence the course of cardiovascular diseases in a dose-response relationship (Fig. 2). However, given the complexity of the cardiovascular system and the large number of causal factors of such diseases, there is no exact mechanism to elucidate the relationship between arecoline and cardiovascular system diseases.
-
When peristalsis occurs in the gastrointestinal tract, it moves and mixes the food to facilitate the absorption of nutrients and water, which is essential for life[6]. Modern research has shown that arecoline, the main active ingredient in areca nut, stimulates the contraction of the gastrointestinal tract muscles in several ways. In the jejunum, arecoline hydrobromide causes smooth muscle contraction by inhibiting voltage-gated potassium channels and inducing smooth muscle cell depolarization[7]. Similarly, in mice and rabbits, arecoline induced colonic smooth muscle motility in a dose-dependent manner, stimulated by the muscarinic (M3) receptor − extracellular Ca2+ influx − Ca2+ store release pathway[80,81]. In addition, as a known antiparasitic with low toxicity, arecoline relieved symptoms of gastrointestinal disorders such as vomiting, diarrhea, and intestinal obstruction caused by the parasites through paralyzing the parasites[82,83].
Regulating intestinal flora
-
Human health is shaped and influenced by the body's commensal microbiota, especially the gut microbiota. For example, one of the mechanisms involved in the pathogenesis of non-alcoholic fatty liver disease (NAFLD) is the alteration of gut microbiota. Zhu et al. found that the abundance of intestinal flora was increased after 0.5−5 mg/kg arecoline treatment in mice, in which Butyricicoccus, Christensenella, and Coriobacteriaceae reversed NAFLD in mice by modulating the Cox-2/PGE2 pathway as well as by increasing the protective effect of intestinal epithelial cells[84]. Additionally, alterations in gut microbiota may induce changes in immunity and exacerbate intestinal disorders. After exposure to 6 and 30 mg/kg arecoline, inflammation and dysbiosis occurred in the intestines of mice, and the results of correlation analyses indicated that arecoline may accelerate the secretion of lipopolysaccharides by promoting an increase in the abundance of the Muribaculaceae bacterium DSM 103720, which in turn encourages the development of inflammation[85]. On a similar note, treatment with 5 mg/kg arecoline decreased the proportion of probiotics in the mouse gut and increased Odoribacter, Bacteroides, and pro-inflammatory factors (IL-6, IL-1β, and TNF-α) as a means of exacerbating ulcerative enteritis in mice[86].
In conclusion, although arecoline can treat dyspepsia, constipation, and anti-parasite, as well as improve the intestinal homeostasis of the NAFLD model through the intestinal flora, inappropriate use of arecoline can disturb the homeostasis of intestinal flora, leading to different degrees of intestinal inflammation and ecological dysregulation. Therefore, despite the medicinal value of arecoline, the mechanism of action on the gastrointestinal tract needs to be further explored in the future, aiming to protect the health of the human gastrointestinal tract while enabling the development of new therapeutic agents and targets for gastrointestinal diseases.
-
The effects of arecoline are systemic, in addition to the impact on organs mentioned above, arecoline also has effects causing bronchoconstriction, hepatotoxicity, reproductive toxicity (Fig. 3). Wang et al. first linked arecoline, Eotaxin-1, and asthma, and their results showed that concentrations of arecoline were negatively correlated with some indexes of respiratory function in asthmatic patients[18]. Arecoline also exhibits toxic effects on hepatocytes and germ cells. After arecoline treatment, hepatocytes in mice showed damage to nuclei and mitochondria, accumulation of sizeable intracellular lipid droplets, decreased expression of antioxidant substances, and an increase in hepatotoxicity markers in a dose-dependent manner[87]. In addition, the testicular weight, sperm count, and viability of male mice were significantly reduced after arecoline treatment[19]; pregnancy rate in female mice was significantly reduced and embryo growth and implantation were affected after 200 μg of arecoline treatment[88]. Interestingly, the hepatotoxicity of arecoline and reproductive toxicity to males were attenuated when supplemented with vitamins C and E, which may be related to the antioxidant function of vitamins C and E[19].
The immune system is an important barrier against external threats and is distributed throughout the body's tissues. For example, arecoline, when present in the host, disrupts this barrier and can lead to various diseases. In the oral cavity and cardiomyocytes, arecoline causes ECM dysregulation by promoting cytokine TGF-β expression, ultimately resulting in fibrosis[24,78]. Similarly, arecoline promotes invasion of OSCC and immune evasion by increasing pro-inflammatory factors and resistance to CD8 T cells[46,47]. In the digestive system, however, arecoline indirectly acts on immunity by altering the gut flora, causing or aggravating gut inflammation[86]. For ease of understanding, we list the effects of arecoline on each of the systems mentioned in this section and their possible mechanisms in Table 2.
Table 2. Other toxicological and pharmacological effects of arecoline.
Effect Animal/cell Specific effect Pathway/mediators Dose Ref. Respiratory system Human and dermal and gingival fibroblast Causing lung function impairment In pro-inflammatory conditions (IL-4 and
TNF-α), arecoline can induce eotaxin-1 release and alter the disease process in asthma25 and 100 μg/mL [18] Human Asthma Possibly related to arecoline-induced bronchoconstriction / [89] Hepatotoxicity Human liver microsome and Male Wistar rats Hepatotoxicity By increasing the hepatic CYP2E1 and CYP2B activity, induced oxidative damage, liver cirrhosis, and hepatocellular carcinoma 4, 20, and 100 mg/kg/d [90] HA22T/VGH hepatoma cells Inducing anoikis By inhibiting STAT3 and SHP2 phosphorylation, decreasing the levels of anti-apoptotic factors, as well as by promoting the activity of pro-apoptotic factors 0−100 μg/mL [91] Human and C57BL/6 mice’s organ of Corti and spiral ganglions Sensorineural hearing impairment Reducing cochlear explant cell activity, inducing cell death and ROS production by causing disruption of hair cells in the organ
of Corti0.2, 0.8, 2, and 10 mM [92] Mice Fatty degeneration and inflammatory infiltration By increasing serum alkaline phosphatase, glutamate oxaloacetate transaminase, glutamate-pyruvate transaminase, and decreasing levels of reduced glutathione, glutathione-S-transferase, SOD, and catalase 10 mg/kg body weight [19] Mice Decreasing nuclear size; the rough endoplasmic reticulum with profusely inflated cisternae and abundance of lipid droplets By Upregulating SGOT and SGPT (hepatotoxicity marker enzymes) in serum 5, 10, and 20 mg/kg body weight [87] Reproduction Zebrafish embryos Reducing survival of embryos with growth retardation and lower heart rate General cytotoxic effects mainly due to intracellular thiol depletion 0.01%−0.04% (wt/vol) [93] Oocyte Apoptosis By disrupting actin filament dynamics, spindle assembly, and kinetochore-microtubule attachment stability, mitochondrial distribution, and increasing oxidative stress levels 180 μg/mL [94] ICR mice and blastocysts Reduction of early embryos and inhibition of blastocyst growth and expansion By inducing DNA damage, cell cycle arrest, or apoptosis 0−8.47 × 10−2 M [88] Male rats Stimulation of testosterone secretion By activating L-type calcium channels, increasing 17β-hydroxysteroid dehydrogenase activity and StAR expression, thereby stimulating testosterone production 1 μg/kg [95] Immunity and endocrine Swiss albino mice Lymphocyte depletion of the thymic cortex and the B and T lymphocyte areas in the spleen and MLN, Elevated corticosterone, SGOT, and SGPT levels, and decreased white and red blood cell counts Not mentioned 20 mg/kg [96] Adult male mice The orientation of nuclei was irregular, follicle degeneration, a decrease in the T3, T4, number, and size of thyroid follicles, and an increase in the TSH level MAChRs mediate the effect of arecoline on thyroid 10 mg/kg [97] BALB/c mice Reducing the spleen index, hemolysin, IL-2 production, and splenocyte proliferation induced by concanavalin A or lipopolysaccharide Mediated via mAChRs 2 mg/kg [98] Fat Mouse 3T3-L1 cells and human Adipocyte dysfunction Inhibiting adipogenic differentiation, inducing adenylate cyclase-dependent lipolysis, and interfering with insulin-induced glucose uptake ≥ 300 µM [99] 3T3-L1 cells Regulating the growth of preadipocytes Inhibiting the CDK family and the CKI pathway by inactivating AMPK activity as
well as the intracellular ROS pathway0−1,000 μM [100] -
This paper provides an up-to-date review of the pharmacologic and toxicologic mechanisms of arecoline. We focus on the oral cavity, central nervous system, cardiovascular system, and digestive system and establish a framework for the pharmacological and toxicological mechanisms of systemic systems after arecoline interventions, hoping to provide some directions for refining the understanding of the mechanism of action of arecoline and its future development. We have found arecoline to have potential therapeutic effects by promoting excitation and improving learning and memory through modulation of nAChRs and mAChRs, as well as causing smooth muscle contractions, promoting intestinal peristalsis, treating indigestion, and being antiparasitic in a way that paralyzes parasites. However, arecoline has been shown to cause varying degrees of damage to various systems throughout the body, including causing OSF, OSCC, neurotoxicity, addiction, cardiotoxicity, hepatotoxicity, and reproductive toxicity. Furthermore, in the effects of arecoline on the CNS and digestive system, we found a dose-effect relationship between arecoline, pharmacology, and toxicology, i.e., low doses are beneficial while high doses are harmful. Therefore, to rationally utilize the pharmacological properties of arecoline, further studies are needed to understand the pharmacological and toxicological mechanisms of arecoline fully and to clarify the dosage-effect relationship and the long-term effects to ensure that the protection of human health and safety accompanies the development of the drug.
This work was supported by the National Natural Science Foundation of China Project (32200387) and the Emergency Project for Risk Assessment of Areca Nut (Key Project of the Department of Agriculture and Rural Affairs of Hainan Province & Wanning Municipal People's Government).
-
The authors confirm contribution to the paper as follows: writing - original draft: Liu H; visualization: Liu H; writing - review & editing: Zheng H, Wang X; supervision: Zheng H, Wang X; resources: Hu X, Chen F, Zheng H, Zhang J; project administration: Hu X, Chen F, Zheng H, Zhang J, Wang X; funding acquisition: Hu X, Chen F, Zheng H, Zhang J, Wang X. All authors reviewed the results and approved the final version of the manuscript.
-
All data generated or analyzed during this study are included in this published article.
-
The authors declare that they have no conflict of interest.
- Copyright: © 2024 by the author(s). Published by Maximum Academic Press on behalf of China Agricultural University, Zhejiang University and Shenyang Agricultural University. This article is an open access article distributed under Creative Commons Attribution License (CC BY 4.0), visit https://creativecommons.org/licenses/by/4.0/.
-
About this article
Cite this article
Liu H, Zheng H, Zhang J, Chen F, Hu X, et al. 2024. Review of the toxic effects and health functions of arecoline on multiple organ systems. Food Innovation and Advances 3(1): 31−41 doi: 10.48130/fia-0024-0005
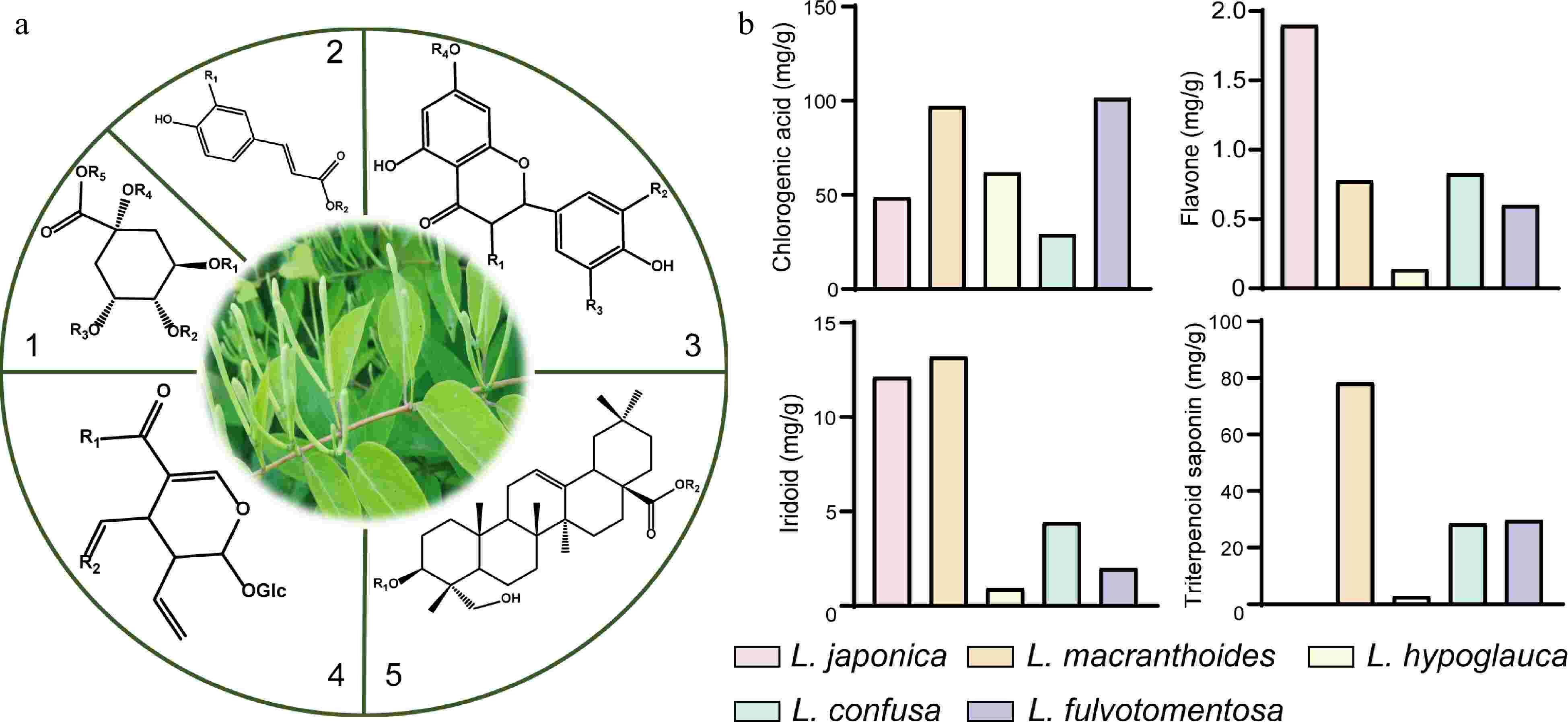