-
The avocado (Persea americana Mill.), is a tropical and subtropical fruit, that originated from Central America and, more specifically, from Mexico, Guatemala, and West India[1]. Avocado is considered a complementary food with high nutritional value and bioactive components that could provide children with all benefits of fruits and vegetables[2]. Fruit suspensions consist of disintegrated vegetable cells and cell wall material dispersed in a fluid comprised of soluble components such as sugars, salts, and acids. However, avocado presents a particular chemical composition, rich in proteins, fibers, and lipids, which can provide rheological information different from other types of fruit pulps[3,4].
Understanding the rheological properties of food products is essential for many industry segments. Rheological data is required for equipment design in extrusion, pumping, mixing, and agitation processes[5]. It is also important in food development and quality control as rheological data is linked to food texture, which is an important factor in food products' acceptability[6]. Finally, the fundamental understanding of food materials structures and interactions through the development of equations for rheological characterization is also an important feature to consider.
Food products and especially fruit fluids typically present non-newtonian shear thinning behavior caused by a complex microstructure originating from a mixture of different components that can be either soluble or suspended[7]. Information about the rheological properties of avocado pulp and avocado pulp products is scarce since most articles focus on avocado oil[8]. The shear thinning behavior of avocado pulp and diluted avocado pulp has been described, revealing a pseudoplastic behavior with viscosity decreasing with avocado pulp concentration[9]. The effects of ultra-high pressure on the flow and viscoelasticity of avocado pulp revealed a shear thinning behavior but a lack of fit under the Herschel-Bulkley model[10]. The effects of ripening on the rheological properties of Avocado pulp revealed a Bingham plastic behavior[11]. More recently, the rheological properties were determined for avocado pulp after being minimally processed, high shear processed, or treated by malaxation, dilution, or sonication. Neither process altered the solid-like behavior of the avocado pulp. However, the storage moduli decreased with the mechanical treatments and water dilution, and both viscoelastic moduli increased with sonication treatment[12].
Maltodextrin, a widely used polysaccharide in the food industry, especially in beverage systems, is essential for its functional properties like thickening, stabilizing, and bulking[2,13]. As a derivative of starch, it enhances mouthfeel and body in beverages without significantly increasing sweetness or altering flavor, making it ideal for health and energy drinks. Its use in avocado-based beverages is particularly intriguing for studying its interaction with avocado's fats and fibers, potentially altering viscosity, consistency, and sensory attributes. This research aims to understand how maltodextrin modulates the rheological and textural properties of avocado pulp, a key factor in developing innovative, functional, and appealing avocado-based beverages in response to growing consumer demand. However, there is a noticeable gap in understanding how avocado pulp interacts with additives like maltodextrin, particularly under varying temperature conditions, which are commonly encountered in industrial processing. Temperature variations can significantly impact the rheological properties of food products, influencing their processing, storage, and sensory attributes.
Given the growing interest in avocado as a food product and its intensive processing in the industry, there is a clear need for more comprehensive rheological studies that take into account the effect of temperature. This research aims to bridge this gap by conducting detailed rheological and textural analyses under different temperature conditions. Specifically, the study seeks to elucidate the complex interactions between avocado pulp and maltodextrin across a range of temperatures, and how these interactions influence the flow properties of the pulp. Understanding the temperature-dependent behavior of these interactions is crucial for optimizing processing techniques and improving the quality of avocado-based food products, ensuring their stability and consistency under various storage and consumption conditions.
-
Avocado (Persea Americana Mill.), were hand picked and bought at a local market. Commercial cow milk was also purchased at local supermarkets. Maltodextrin DE10 Corn Brazil was used as drying additive.
Avocado pulp preparation
-
Avocado skin and kernel were hand removed and separated from the pulp. Then the five formulations were prepared as described in Table 1. Initially, the avocado was washed with abundant water, and then the bark and core were removed. The avocado pulp was placed in a knife homogenizer along with the desired amounts of water and maltodextrin. Each formulation was homogenized for 10 min. No other additives were used. Maltodextrin amounts were chosen to cover a wide range of concentrations.
Table 1. Tested avocado formulations and respective chemical composition.
Formulations Unit SM0 SM5 SM10 SM20 SM40 Avocado g 450 450 450 450 450 Water g 1000 1000 1000 1000 1000 Maltodextrin g 0 75.5 151 302 604 Chemical composition Water g/100 g 91.40 ± 0.12 86.89 ± 0.21 82.72 ± 0.20 75.62 ± 0.17 64.53 ± 0.19 Protein g/100 g 0.61 ± 0.007 0.59 ± 0.004 0.55 ± 0.002 0.50 ± 0.002 0.42 ± 0.001 Lipids g/100 g 4.78 ± 0.005 4.61 ± 0.005 4.33 ± 0.003 3.95 ± 0.005 3.35 ± 0.003 Ash g/100 g 0.52 ± 0.008 0.46 ± 0.0007 0.47 ± 0.001 0.45 ± 0.002 0.38 ± 0.005 Carbohydrates g/100 g 2.68 ± 0.005 7.52 ± 0.05 11.86 ± 0.04 19.45 ± 0.04 31.31 ± 0.03 Rheological measurements
-
Viscosity and torque measurements were made on avocado mixtures using a Brookfield viscometer (RV+ model, Brookfield Engineering Laboratories Inc., MA, USA) at temperatures of 5, 25, and 35 °C with nine spindle speeds (1, 3, 5, 10, 20, 30, 50, 60, and 100 rpm). The temperature was maintained using a thermostatically controlled water bath. All data were taken after 30 s in each sample, with a rest in time between the measurements at different spindle speeds. Appropriate spindles (spindle no: 3 or 4) were used to get all readings within the scale. A 500 ml beaker was used for all measurements with the guard leg on and enough samples were added to just cover the immersion grooves on the spindle shafts. All experiments were replicated three times. Average shear stress and shear rates were calculated using the method of Mitschka[14].
Texture analysis
-
Instrumental texture measurements were performed using a penetrometer (TA-TX plus, Stable Micro Systems, UK) equipped with a 50 N load cell. An A/BE-d35 probe was compressed twice against each bread sample of 25 mm to a defined depth (50%) at a rate of 1.7 mm/s. Measurements were performed in triplicate. As a result of these experiments, force–time curves were built and analyzed to determine the average of mechanical parameters (Firmness, Cohesiveness, Consistency and Viscosity index). The firmness parameter is related to the force required to attain a given deformation and is represented by the altitude of the first peak on the force-time curve. The cohesiveness parameter is related to the ability of the sample to resist deformation and maintain its structure. It is calculated as the ratio of the area under the force-time curve produced in the first compression to the area under the curve produced in the second compression. The consistency parameter is related to the resistance of the sample to deformation and is determined by analyzing the force-time curves. One common method is to calculate the area under the curve, which represents the work done on the sample during the test. A larger area under the curve typically indicates a higher consistency, as it suggests that more work is required to deform the sample. The viscosity index is a parameter related to the flow behavior of the sample under deformation. It is calculated as the ratio of the area under the force-time curve to the maximum force applied[15].
Data modeling
Rheological models
-
The term Bingham fluid typically represents a fluid with a yield stress and then a Newtonian behavior. Bingham fluid model is presented in Eqn (1):
τ+τ0=Kγ (1) where, τ is the shear stress (Pa), τ0 is the yield stress (Pa), K the Bingham consistency index and γ is the shear rate (s−1). Ostwald-Waelle rheological model, or power law equation oss presented in Eqn (2).
τ=Kγn (2) where, K is the consistency index (Pa·sn) and n is the flow behavior index.
The apparent viscosity can be correlated with the shear rate through the adapted Cross model as presented in Eqn (3).
η=η∞+η0−η∞(1+(αc⋅γ)m≈η0(1+(αc⋅γ)m (3) where, η the apparent or effective viscosity (Pa·s),
is the infinite-shear-rate-viscosity,η∞ is the zero-shear-rate-viscosity αC is the Cross time constant (s), m the Cross exponent. Sinceη0 values are much higher thenη0 , the model was modified.η∞ Thermodynamic models for flow
-
Fluid flow is kinetically controlled so that an Arrhenius-type equation can be written:
ηapp=Aexp(EaR⋅T) (4) where, ηapp is the apparent viscosity (Pa·s), A is a frequency factor associated with molecular collision rate (Pa·s), Ea is the activation energy (kJ/mol). R is the perfect gas constant (kJ/K mol) and T is the absolute temperature.
Statistical analysis
-
All measurements were performed in triplicate. The results were evaluated by one-way analysis of variance (ANOVA) and average comparison by Tukey's test at 5% probability. All models presented significance relevance for a p < 0.05. Statistical work was performed using STATISCA 12.0, Statsoft, Tulsa, OK, USA.
-
Rheological properties are essential for many industrial features and quality control but are also important to better understand the molecular interactions within the liquid material. Figure 1 presents the viscosity curves of all prepared samples at three different temperatures. All samples revealed thixotropic (shear thinning) properties throughout the entire range of shear rates used, revealing a non-Newtonian behavior since shear rate increases with shear stress, which can be generally explained by a mixture of dissolved and suspended compounds. This result agrees with other works using avocado, once Tabilo-Munizaga and co-workers also detected a shear thinning behavior for avocado puree[10]. However, from a rheological perspective, water, a main component of our formulation, is a Newtonian fluid. The rheology of these fluids is influenced by several parameters, being the most important the inter-particle forces. The shear thinning effect can be generally explained by asymmetric molecules aligned in shear planes, thereby reducing frictional resistance[16]. The presence of fat globules from avocados can significantly contribute to the shear thinning effect. Applying shear stress can progressively disrupt fat globules creating more agglomerates with smaller volumes and less interstitial volume. Therefore, reducing the fat volume fraction leads to less apparent viscosity explaining the shear-thinning behavior.
Temperature plays a significant role in influencing the viscosity of substances, including avocado pulp. The observed reduction in viscosity from 4 to 37 °C can be attributed to several factors, such as increased molecular motion and changes in the properties of fat globules and their interactions. As temperature rises, molecules move more rapidly, weakening intermolecular interactions like hydrogen bonding and van der Waals forces[17]. This leads to a decrease in viscosity as the substance flows more easily. In addition to molecular motion, the behavior of fat globules and other components like polysaccharides within avocado pulp can also be affected by temperature changes. As temperature increases, fat globules become more fluid and their interactions alter, contributing to the reduction in viscosity. Moreover, higher temperatures can cause changes in the conformation and solubility of polysaccharides and other components, impacting the overall viscosity of the pulp. Understanding these temperature-related changes is crucial for applications such as food processing and product development, as they can affect the final product's texture, stability, and quality.
While maltodextrin is not typically considered an effective emulsifier, its amphiphilic nature and interaction with other components in a mixture can still influence the rheological properties of the system. In the case of the avocado-based samples, adding small amounts of maltodextrin led to a decrease in apparent viscosity, as shown in Fig. 2. This observation suggests that maltodextrin may interact with the fat globules in the avocado pulp, potentially causing some disruption in the globules or altering the balance between repulsive and attractive forces. However, it is essential to note that these effects may be limited and depend on the maltodextrin concentration. In the study, the apparent viscosity only started to increase after the maltodextrin concentration reached 20%. This increase in viscosity could be attributed to other factors, such as the thickening properties of maltodextrin or increased interactions between maltodextrin and other components in the system at higher concentrations. In summary, while maltodextrin is not a strong emulsifier, its presence, and interactions with other components, such as fat globules in avocado pulp, can impact the rheological properties of the mixture. The effects of maltodextrin on viscosity and emulsion stability may be concentration-dependent and should be considered when formulating food products containing both maltodextrin and avocado. This insight could be useful for optimizing products containing avocado and maltodextrin formulation, considering the desired rheological properties for specific applications.
Figure 2.
Effect of maltodextrin concentration on the apparent viscosity of diluted avocado pulp at 10 s−1 shear rate.
Bingham flow modeling
-
Natural fluids require a critical level of stress to initiate flow. Below this critical value, fluids behave as solids absorbing stress without movement[18]. After reaching the critical level, the fluid yields to flow, hence the term yield stress. To determine the yield stress of the solutions and the impact of maltodextrin concentration, bingham model was fitted to the experimental data and the results summarized in Table 2. Yield stress values ranged from 24.84 to 12.71 and are influenced by temperature and maltodextrin concentration. Similar values for yield stress were observed for different fruit purees[19]. Moreover, yield stress values are higher for diluted avocado at 0% concentration of maltodextrin, and only after 20% of maltodextrin the yield stress surpass that initial value. Thus, small percentages of maltodextrin seem to reduce the initial flow barrier and act as a lubricant. At 25 and 37 °C, the initial flow barrier is similar for all maltodextrin concentrations, and only at the concentration of 40% is this value higher than the previous formulations. Yield stress reveals a three-dimensional structure that prevents flow at low shear rates. When higher shear rates are applied, the hydrodynamic forces disrupt secondary bonds such as ionic interactions and hydrogen bonds. Therefore, results can be explained by the formation of such a three-dimensional structure that firstly is interrupted by maltodextrin at low concentration but then, after a critical concentration, forms a new macromolecular structure. This is quickly identified at low temperatures because the density of secondary bonds is temperature dependent.
Table 2. Parameters determined for Bingham and Ostwald-Waelle flow model, and Cross Viscosity Model.
Samples Temperature (°C) τ0 (Pa·s) K (Pa·s) R2 K (Pa·sn) n R2 η0 (Pa·s) αC (s) γC (s−1) m R2 SM0 4 18.26 ± 0.15 0.057 ± 0.002 0.9709 8.764 ± 0.1 0.22 ± 0.01 0.9985 1.1E+03 ± 100 1145 ± 115 8.7E-04 ± 0.0001 0.8 ± 0.08 0.9986 25 13.17 ± 0.12 0.060 ± 0.003 0.9956 5.681 ± 0.08 0.27 ± 0.01 0.9995 1.4E+04 ± 1400 1486 ± 149 6.7E-04 ± 0.00007 0.86 ± 0.086 0.9965 37 13.00 ± 0.10 0.054 ± 0.002 0.9833 5.819 ± 0.07 0.25 ± 0.01 0.9966 9.4E+03 ± 940 4450 ± 445 2.2E-04 ± 0.00002 0.75 ± 0.075 0.9987 SM5 4 16.04 ± 0.18 0.095 ± 0.004 0.9839 6.503 ± 0.09 0.29 ± 0.01 0.9998 5.2E+03 ± 520 3661 ± 366 2.7E-04 ± 0.00003 0.70 ± 0.07 0.9998 25 13.75 ± 0.13 0.044 ± 0.002 0.9896 6.508 ± 0.08 0.23 ± 0.01 0.9965 2.4E+04 ± 2400 9180 ± 918 1.1E-04 ± 0.00001 0.77 ± 0.077 0.9996 37 11.96 ± 0.11 0.057 ± 0.003 0.9910 5.135 ± 0.06 0.27 ± 0.01 0.9948 2.2E+04 ± 2200 15803 ± 1580 6.3E-05 ± 0.000006 0.75 ± 0.075 0.9970 SM10 4 16.88 ± 0.14 0.105 ± 0.004 0.9886 6.738 ± 0.1 0.31 ± 0.01 0.9986 1.7E+03 ± 170 22939 ± 2294 4.4E-05 ± 0.000004 0.69 ± 0.069 0.9983 25 13.13 ± 0.12 0.071 ± 0.003 0.9784 5.452 ± 0.07 0.29 ± 0.01 0.9936 1.2E+03 ± 120 6864 ± 686 1.5E-04 ± 0.000015 0.75 ± 0.075 0.9993 37 12.94 ± 0.09 0.062 ± 0.003 0.9717 5.578 ± 0.06 0.27 ± 0.01 0.9953 9.9E+03 ± 990 4938 ± 494 2.0E-04 ± 0.00002 0.75 ± 0.075 0.9993 SM20 4 21.38 ± 0.20 0.179 ± 0.005 0.9932 7.831 ± 0.11 0.35 ± 0.01 0.9975 1.4E+04 ± 1400 24449 ± 2445 4.1E-05 ± 0.000004 0.66 ± 0.066 0.9991 25 12.71 ± 0.11 0.063 ± 0.002 0.9915 5.393 ± 0.07 0.31 ± 0.01 0.9920 1.2E+04 ± 1200 17054 ± 1705 5.9E-05 ± 0.000006 0.72 ± 0.072 0.9894 37 13.08 ± 0.10 0.047 ± 0.002 0.9914 6.081 ± 0.08 0.27 ± 0.01 0.9917 2.8E+04 ± 2800 14109 ± 1411 7.1E-05 ± 0.000007 0.77 ± 0.077 0.9994 SM40 4 24.84 ± 0.25 0.472 ± 0.01 0.9946 7.429 ± 0.12 0.48 ± 0.02 0.9991 3.7E+03 ± 370 41626 ± 4163 2.4E-05 ± 0.000002 0.53 ± 0.053 0.9989 25 19.41 ± 0.18 0.314 ± 0.008 0.9874 6.026 ± 0.09 0.46 ± 0.02 0.9976 6.3E+03 ± 630 131529 ± 13153 7.6E-06 ± 0.00000076 0.54 ± 0.054 0.9961 37 17.28 ± 0.15 0.248 ± 0.007 0.9872 5.509 ± 0.07 0.44 ± 0.02 0.9975 6.4E+03 ± 640 42249 ± 4225 2.4E-05 ± 0.000002 0.59 ± 0.059 0.9632 Additionally, when small percentages of maltodextrin are introduced, the fat globules may be affected by the altered balance of attractive and repulsive forces between the globules themselves and the maltodextrin molecules. The disruption of fat globules at low concentrations of maltodextrin could explain the reduced initial flow barrier and the lubricating effect observed. As the concentration of maltodextrin increases, it may lead to a new macromolecular structure that interacts with the fat globules and other components, causing an increase in yield stress.
Furthermore, temperature changes can impact the behavior of fat globules, affecting their fluidity and interactions within the system. Fat globules are more likely to maintain their structure at lower temperatures, leading to a higher density of secondary bonds and more pronounced yield stress. As the temperature increases, fat globules become more fluid, reducing the strength of these secondary bonds and lowering the yield stress. Bienvenue et al. identified increases in yield stress when concentrated milk was stored for more extended periods due to the formation of more secondary bonds between milk molecules[20]. Consistency index values vary between 0.054 to 0.472 Pa·s, generally increasing with maltodextrin concentration and decreasing with temperature.
Ostwald-Waelle flow
-
To better understand the deviation from the Newtonian fluids, the experimental data was fitted with the Ostwald Waelle (Power law equation). Results for both parameters are summarized in Table 3. The flow curves generated by the data fitting are presented in Fig. 3. From Table 2, considering that the R2 values are all above 99%, it is concluded that the power law model fits well the experimental data. Consistency indexes are similar to the constant dynamic viscosity of Newtonian fluids and the obtained values ranged from 5.135 to 8.764 Pa·sn. Accordingly, to Rao and co-workers, these values can be considered high, and typical of purees with high solid concentrations[21]. The effect of maltodextrin concentration is not clear since values can be considered constant throughout the entire series of concentrations. Even comparing the SM0, which has no maltodextrin, with the others, no clear differences are identified. However, with temperature, it is possible to identify higher values at 4 °C, but similar values at 25 and 37 °C. Higher consistency indexes do not necessarily guarantee a fluid with higher viscosity because this property also depends on the flow behavior and shear rate for shear-thinning fluids. For a solution to be considered a Newtonian fluid, the flow index value should be 1 as viscous stress and strain rate would be related by a constant dynamic viscosity (τ = μ·γ). However, the power law equation fitted to the experimental data gave values for flow index ranging from 0.222 to 0.436, meaning that all studied solutions were non-newtonian. Since the flow index is lower than 1, all samples presented shear-thinning properties where increasing shear rate results in lower apparent viscosity or higher stress is required to flow (Fig. 2). The more the flow index is close to 1 the less shear thinning the sample is. Similar values of consistency index and flow behavior where obtained for mango and pineapple pulps[22]. Thus, analyzing Table 2, it is clear that maltodextrin concentration have small impact on the flow index since values are almost constant until 20%. Above 20% the flow index suffers a sharp increase for all temperatures, meaning that fluid is less shear thinning effect. This can be explained by an overlap between the hydrodynamic domains of the maltodextrin coils. Once a given shear rate is reached, such maltodextrin coils start to disentangle and become aligned on the shear rate plane and interact via hydrogen bonds in a similar fashion to starch retrogradation. However, because the concentration has exceeded the critical concentrations, the disentanglements are less pronounced, and thus the shear thinning is also less pronounced. Similar behavior was detected on oil/water emulsions containing tapioca maltodextrin, where increasing concentrations of maltodextrin resulted in less shear thinning fluids[23]. It is also known that maltodextrin solutions are Newtonian fluids.
Table 3. Arrhenius-type equation parameters for apparent viscosity of diluted avocado pulp samples.
Activation energy (kJ/mol) A (Pa·s) R2 SM0 5.96 ± 0.6 1.8E-2 ± 0.0016 0.9635 SM5 7.35 ± 0.33 1.1E-2 ± 0.0023 0.9734 SM10 8.32 ± 0.65 7.52E-3 ± 0.00049 0.9798 SM20 12.93 ± 0.34 2.25E-4 ± 0.0000345 0.9666 SM40 10.36 ± 0.23 8.23E-3 ± 0.000431 0.9943 Figure 3.
–Flow curves for avocado formulations at (a) 4 °C, (b) 25 °C, and (c) 37 °C modelled using Ostwald-Waelle models.
Cross model
-
Cross model has been used to evaluate the rheological properties of polysaccharides where coil entanglements are present[24]. Shear thinning dispersions often exhibit three stages of viscous response when sheared at a wide range of shear rates. At low shear rates, fluids tend to present Newtonian properties with a zero-shear-constant
viscosity that is followed by a second stage, that is the typical shear thinning range where the viscosity drops as a function of the shear rate. At the third stage, fluids reach a constant infinite-shear-rate viscosity.η0 Results for all parameters are presented in Table 2. Zero-shear-viscosity ranged between 103 to 104 with no clear trend for any of the parameters used. The reciprocal of cross time constant, (αC = 1/γC ) gives the order of magnitude of the critical shear rate that marks the end of the Newtonian plateau region and the beginning of the shear thinning region. Values ranged from 10−4 for lower concentrations and 10−5 for higher maltodextrin concentrations. The difference between the lower and higher concentrations lies on the rearrangements of macromolecules. Macromolecules do not need to rearrange or align at lower shear rates due to flow, but at stage 2, medium shear rates make molecules re-arrange, causing the shear thinning effect. Because the concentration of macromolecules is higher, the onset of the shear thinning effect occurs at smaller shear rates. The values of m are the cross exponent, which are m = (1 − n), where n is the power law flow behavior. Therefore, decreasing m values means that fluid is less shear-thinning and more Newtonian. Similarly to the power law model, flow behavior values are within the same range (0.8 to 0.7) for all concentrations and temperatures except when maltodextrin concentration is higher than 20%, where the flow falls for close to 0.5. Lira-Ortiz and co-workers found much lower values for the parameters of the cross model when analyzing the rheological properties of pectin from prickly pears. The smaller values might be due to the lower concentrations used[25].
Thermodynamic analysis of avocado pulp flow behavior
-
Fluid flow is kinetically controlled, so an Arrhenius-type equation can determine the viscosity at any given temperature as long as the activation energy is determined. Equation 4 is thermodynamically derived and assumes the free volume theory, where an energy barrier exists for a molecule or a group of molecules, to move from one position to another, before and after flowing.
Since Ea expresses the difficulty for a molecule to move from one position to another the intensity of molecular interactions, the bulkiness and rigidity of side groups are parameters that influence the Ea. Table 3 summarizes the activation energy of all samples. Values ranged between 5.96 to 12.93 kJ/mol depending on maltodextrin concentration. Shear rate used for calculation was 100 s−1 since it is considered as a typical value for pipe flow, agitation or swallowing[26]. Similar results were found for acai blueberry pulp, where increases in Brix resulted in higher activation energy[27]. The activation energy is the energy required to form a molecular cavity where to a given molecule 'jumps' leaving its temporary equilibrium position. The increases in the activation energy with maltodextrin concentration suggest that the hydrocolloid forms a new structure within the avocado pulp, hindering the mobility of molecules. The frequency factor is often associated with molecular collision, and the obtained values tend to decrease with increasing maltodextrin concentration. The explanation for such a result might be the molecular confinement and reduced mobility and, thus, reduction in the collision rate.
Texture properties
-
We examined the effects of varying maltodextrin concentrations and temperatures on the textural properties of an avocado pulp. The textural parameters investigated include cohesiveness, consistency, firmness, and viscosity index and results are presented in Table 4.
Table 4. Instrumental texture analysis of avocado pulp samples with different maltodextrin concentrations at different temperatures.
Maltodextrin
concentrationTemperature
(°C)Cohesiveness Consistency
(N)Firmness
(N)Viscosity
index (Pa·s)0 4 0.22 ± 0.01 7.81 ± 0.05 0.35 ± 0.02 0.47 ± 0.03 0 25 0.18 ± 0.01 6.97 ± 0.04 0.33 ± 0.02 0.31 ± 0.02 0 37 0.16 ± 0.01 5.69 ± 0.03 0.27 ± 0.01 0.17 ± 0.01 5% 4 0.22 ± 0.01 8.06 ± 0.05 0.36 ± 0.02 0.42 ± 0.03 5% 25 0.18 ± 0.01 7.52 ± 0.04 0.34 ± 0.02 0.37 ± 0.02 5% 37 0.17 ± 0.01 6.25 ± 0.03 0.29 ± 0.01 0.22 ± 0.01 10% 4 0.24 ± 0.01 8.14 ± 0.05 0.38 ± 0.02 0.44 ± 0.03 10% 25 0.19 ± 0.01 7.53 ± 0.04 0.35 ± 0.02 0.37 ± 0.02 10% 37 0.18 ± 0.01 7.29 ± 0.04 0.32 ± 0.01 0.27 ± 0.01 20% 4 0.32 ± 0.02 10.72 ± 0.06 0.42 ± 0.02 0.79 ± 0.04 20% 25 0.19 ± 0.01 7.82 ± 0.04 0.35 ± 0.02 0.35 ± 0.02 20% 37 0.17 ± 0.01 6.94 ± 0.04 0.35 ± 0.02 0.19 ± 0.01 40% 4 0.34 ± 0.02 12.09 ± 0.07 0.58 ± 0.03 0.99 ± 0.05 40% 25 0.28 ± 0.02 11.72 ± 0.06 0.57 ± 0.03 0.76 ± 0.04 40% 37 0.23 ± 0.01 8.75 ± 0.05 0.42 ± 0.02 0.42 ± 0.02 Cohesiveness, a measure of the internal binding forces within the food matrix, generally increased with maltodextrin concentration across all temperatures. This can be attributed to the fact that maltodextrin acts as a bulking agent and thickener, improving the overall structure and binding within the pulp. Additionally, cohesiveness was found to be highest at the lowest temperature (4 °C), which could be due to the increased viscosity at lower temperatures, leading to stronger interactions between the avocado pulp and maltodextrin particles.
Consistency, an indicator of the thickness and flow behavior of the avocado pulp, also increased with maltodextrin concentration. This can be explained by the water-binding capacity of maltodextrin, which reduces the free water content and thus increases the thickness of the avocado pulp. The consistency was highest at 4 °C, followed by 25 °C, and then 37 °C. This trend can be attributed to the temperature-dependent behavior of the avocado pulp and maltodextrin-water interactions, where lower temperatures result in increased viscosity and reduced flow.
Firmness, a measure of the force required to deform the avocado pulp, increased with maltodextrin concentration, consistent with the enhancement of the structural integrity of the avocado pulp due to the presence of maltodextrin. The firmness was highest at 4 °C, decreasing as temperature increased. This could be due to the avocado pulp's temperature sensitivity and the maltodextrin's water-binding capacity, which tends to weaken at higher temperatures.
The viscosity index, which reflects the resistance of the avocado pulp to flow, increased with maltodextrin concentration, suggesting that the presence of maltodextrin increased the overall viscosity of the avocado pulp avocado pulp. The viscosity index was highest at 4°C, decreasing as the temperature increased. This is likely because viscosity generally decreases with increasing temperature as the components' molecular motion and kinetic energy increase, reducing the resistance to flow.
-
In conclusion, this study comprehensively examined the rheological and textural properties of an avocado pulp with varying maltodextrin concentrations and temperatures. From a rheological perspective, our results highlighted that the avocado pulp exhibits non-Newtonian, shear-thinning behavior, with the Bingham, Ostwald-Waelle, and Cross models fitting the experimental data well. Maltodextrin concentration had a discernable influence on these properties, generally acting to reduce the initial flow barrier and serve as a lubricant at lower concentrations, while increasing yield stress and viscosity at higher concentrations. The thermodynamic analysis further reinforced these findings, demonstrating a direct relationship between maltodextrin concentration and activation energy, suggesting that maltodextrin forms a new structure within the avocado pulp that influences molecular mobility. Texturally, the avocado pulp's cohesiveness, consistency, firmness, and viscosity index increased with maltodextrin concentration, signifying the role of maltodextrin as a bulking agent and thickener. Furthermore, temperature pronounced affected both rheological and textural properties, with lower temperatures resulting in increased yield stress, viscosity, cohesiveness, consistency, and firmness. These findings have significant implications for the food industry, particularly when formulating fruit-based beverages with optimized sensory and rheological characteristics. By manipulating maltodextrin concentration and temperature, such beverages' flow behavior and texture can be tailored to suit specific consumer preferences and functional requirements. Further research would be beneficial to understand the behavior of other hydrocolloids and their interactions with fruit pulp in such systems, which would open up new avenues for innovation in the food and beverage industry.
-
The authors confirm contribution to the paper as follows: investigation: Ribeiro S, Lima J, Batista L; formal analysis: Nascimento A; supervision: Almeida R; original draft preparation, review & editing: Lisboa H. All authors reviewed the results and approved the final version of the manuscript.
-
The datasets generated during and/or analyzed during the current study are available from the corresponding author on reasonable request.
-
The authors declare that they have no conflict of interest.
- Copyright: © 2024 by the author(s). Published by Maximum Academic Press on behalf of Nanjing Agricultural University. This article is an open access article distributed under Creative Commons Attribution License (CC BY 4.0), visit https://creativecommons.org/licenses/by/4.0/.
-
About this article
Cite this article
Ribeiro S, Nascimento A, Lima J, Batista L, Almeida R, et al. 2024. Modulation of rheological and textural properties in avocado pulp: impact of maltodextrin concentration and temperature. Food Materials Research 4: e014 doi: 10.48130/fmr-0024-0005
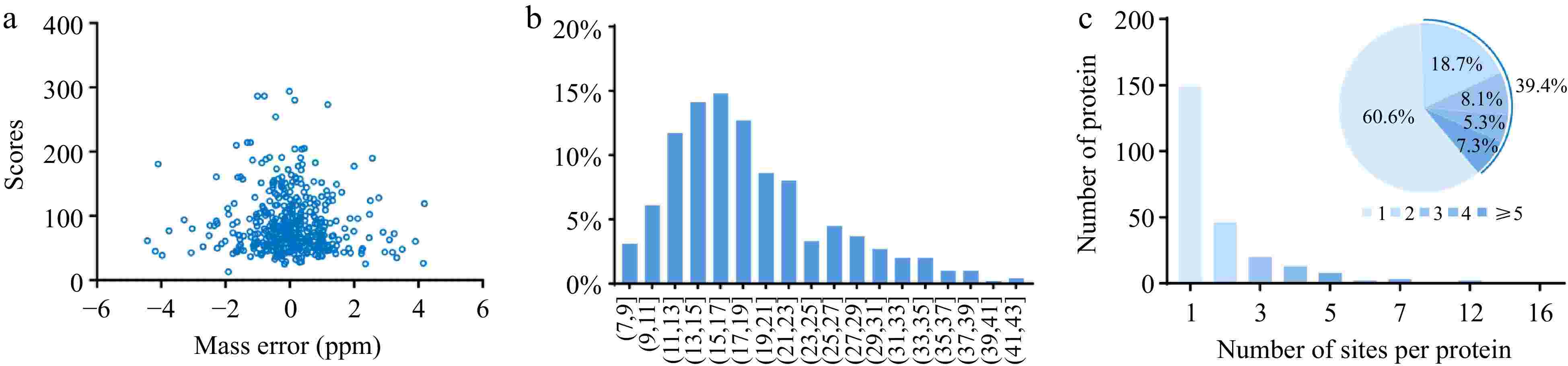