-
Plant polyphenols, organic molecules characterized by phenolic hydroxyl groups, are secondary metabolites mainly synthesized through the pentose phosphate pathway, shikimic acid, and phenylpropionic acid pathways[1,2]. Plant polyphenols with phenol as the basic skeleton are categorized into five categories according to the chemical structure of the carbon atom skeleton structure: lignans, phenolic acids, flavonoids, stilbenes, and other polyphenols[3−5]. They are abundant in nature from a variety of sources, for instance, tannic acid (TA), tea polyphenol (TPs), hesperidin, and chlorogenic acid are rich in grapes, tea, citrus, and coffee beans, respectively[6−8]. According to Grand View Research website analysis, the global polyphenol market size was USD
1.68 billion in 2022 and is expected to grow at a compound annual growth rate (CAGR) of 7.4% from 2023 to 2030[9]. However, many plant polyphenols are still being wasted, such as flavonoids in citrus peels, tea polyphenols in old tea raw materials, apple polyphenols in apple deep-processed waste, etc. In the context of respect for nature, the application of plant polyphenols has attracted much attention, which will undoubtedly continue to expand the plant polyphenol industry[10,11].$ The wide range of biological properties of plant polyphenols, including antibacterial, antioxidant, anti-inflammatory, anticarcinogenic, and improvement of cardiovascular disease impacts, has also expanded their application in the food industry, healthcare, and other fields[12−16]. In addition to diverse biological functions, natural polyphenols have unique physicochemical properties that allow them to bind to various substances through hydrogen bonding, hydrophobic interactions, and π-π interactions[17]. In recent years, plant polyphenols have been transformed from direct application to constructing functional materials using polyphenols as backbone molecules[18]. For example, self-assembly by oxidative self-polymerization of polyphenols[2], construction of metal-polyphenol networks (MPNs) by chelating polyphenols with metal ions[19,20], construction of polyphenol delivery systems by binding to liposome[21], and combination of polyphenols with chitosan, proteins, and other substances to produce hydrogels and coating films[22,23] (Fig. 1).
Currently, research on the application of plant polyphenols is mainly in the medical field, mostly focusing on natural antimicrobial properties. This could tackle the health threat of bacterial resistance and fight the evolving resistant bacteria[24]. Because of the extremely complex microenvironment of drug-resistant bacterial infections, nanomaterials with both bactericidal and immuno-modulating activities are undoubtedly the ideal modality for overcoming drug resistance[25]. Numerous studies have demonstrated the antimicrobial activity of various plant polyphenols[26], such as proanthocyanidins in persimmons, which have antibacterial activity against Staphylococcus aureus (S. aureus) by inducing morphological damage to cell membrane permeability and disrupting membrane integrity[27], phloretin polyphenols in apples regulate the activity of curl genes involved in biofilm formation, inhibiting biofilm formation in Escherichia coli (E. coli)[28], and tea polyphenols inhibit bacterial DNA rotamase and block nucleic acid synthesis for antibacterial purposes[29]. Preparation of nanomaterials using these natural polyphenols holds promise for tackling bacterial drug resistance.
Plant polyphenols also have numerous beneficial effects on health, including preventing many diseases, such as cancer, diabetes, arthritis, cardiovascular disease, and obesity[30,31]. However, they also have some health risks. For example, high concentrations of polyphenols may be toxic to cells by inducing oxidative stress[32], and excessive tea polyphenols can inhibit the activity of sulfotransferases involved in drug metabolism, thereby interfering with the metabolic process of the drug and triggering toxic side effects[33]. Therefore, chemical structural modifications of polyphenols to avoid these risks and improve bioavailability are urgently needed. Emerging nanobiotechnology can construct polyphenols and different ligands into biomaterials with high stability and high bioavailability, and have synergistic effects, expanding the application of plant polyphenols in different fields. Given this, this review aims to provide an overview of plant polyphenol-based biomaterials across diverse disciplines, including nanoparticles, micellar systems, hydrogels, and coating films, indicating their unique functionalities. Moreover, we provide an outlook on the future research directions for plant polyphenol-based biomaterials, aiming to stimulate further innovative applications of plant polyphenols in various fields.
-
Natural polyphenols can undergo self-polymerization, resulting in the formation of natural polyphenol nanodot materials through covalent oxidative polymerization and physical assembly (Fig. 2a)[34]. Compared with monomer gallic acid (GA), the multiple chemical functional groups on the curved surface of GA nanodots can bind more proteins, thereby inhibiting the process of Aβ1-42 fibrosis. The polyphenol nanodots exhibit both good biocompatibility and free radical scavenging ability and are capable of effectively removing Aβ1-42 protein content from the mouse brain, thereby alleviating the symptoms of oxidative stress in mice. Lin et al.[35] prepared mesoporous catechin nanoparticles with an approximate diameter of 100 nm, and mesopores with a diameter of about 15 nm, which were achieved by an effective balance between hydrogen bonding and π-π stacking forces (Fig. 2b). The high surface area of 106 m2/g was found to be capable of efficiently adhering to the surface of Methicillin-Resistant S. aureus (MRSA), causing bacterial surface folds and damage, and 25 μg/mL was sufficient to inhibit the growth of MRSA in vitro completely. In an in vivo wound model, the wound area treated with mesoporous catechin nanoparticles had been reduced by 90% by day 6. The results showed that the synergistic effect of the mesoporous structure and catechin molecules, which interfere with bacterial metabolism, endowed the materials with excellent antimicrobial properties, and endothelial cell growth and angiogenesis were unaffected during the promotion of wound healing.
Figure 2.
(a) Natural polyphenolic ND synthesis through covalent oxidative polymerization and physical assembly pathway[34] ©2023, Wiley. (b) Schematic diagram for the synthesis of mesoporous catechin nanoparticles, structural features, and strong adhesion to bacteria[35] ©2024, ACS Publications. (c) Schematic illustration of composite nanoparticles bound to acidification hyaluronic acid and MMP-targeting peptides[37] ©2024, ACS Publications. (d) Fabrication of the CEPG/CAT-MN patch and its application in the prevention and treatment of RISI[38] ©2024, ACS Publications.
Compared to single polyphenol fractions, co-assembled nanoparticle alkaloids with polyphenols as well as co-assembled binary polyphenols can act synergistically to achieve multiple biomedical functions[36]. Zhang et al.[37] prepared resveratrol and procyanidin as nanoparticles with hyaluronic acid as the core grafted with MMP-targeting peptides (Fig. 2c). This solved the problem of poor stability of the two polyphenols in vivo, and the MMP-targeting peptides achieved regional delivery at the site of injury. NPs can be used as circadian rhythm-dependent myocardial ischemia-reperfusion injury protection drugs. Integration of two polyphenols significantly scavenges reactive oxygen species (ROS), attenuates the inflammatory response in injured cardiomyocytes, and activates the rhythm gene Sirt1, providing a more effective therapeutic effect during the time of day when myocardial ischemia-reperfusion damage is most severe. Also combining two plant polyphenols, Hu et al.[38] co-assembled two natural plant polyphenols, epigallocatechin gallate (EGCG) and curcumin (CUR), into nanoparticles (CEPG) through intermolecular interactions, and CEPG was doped with catalase (CAT) into PVP to prepare a microneedle patch (CEPG/CAT-MN) (Fig. 2d). Adoption of natural compound co-assembly strategy, which improves their bioavailability and reduces toxicity, also can be obtained nanoparticles of different sizes[39]. The experimental results showed that the decomposition of CEPG, which can be triggered by ROS generated in radioactive skin injury (RISI) releases EGCG and CUR. The cascade effect of EGCG and CAT gradually converts superoxide anion into water, thereby mitigating cell damage during the treatment process, while CUR addresses the management of skin inflammation. In conclusion, plant polyphenols utilize multiple forces for self-assembly, transforming polyphenol monomers into supramolecular structures, endowing polyphenols with unique microscopic nanostructures, and imparting effective drug delivery.
-
TA may be the most used plant polyphenol nowadays, its multiple phenolic hydroxyl structures can be complex with a variety of metal ions, in the reduction of toxic side effects of metal ions, it also can enhance the antibacterial activity[40]. Chen & Tang[41] prepared a packaging biomass film by wrapping chitin nanocrystals (CNC) and sodium alginate with FeIII-TA MPN. The notable color changes are caused by the shift in the FeIII and TA composition ratio due to the change in pH value (Fig. 3a). The film has photothermal response antibacterial properties and triggers TA to release antibacterial agents in the pH 4−6 range, realizing real-time visual monitoring of food quality. Similarly, FeIII-TA MPN is on the surface of TiO2 nanospike and combined with antimicrobial peptides can construct a multifaceted nano-coating. Through physical/photothermal/chemo triple-synergistic therapy, the antibacterial rate in vitro and in vivo reached more than 95%. The infection problem caused by titanium and its alloys, which lack antibacterial properties and orthopedic implants was solved[42]. Furthermore, the good photothermal effect of MPN can significantly improve the antimicrobial effect. Ye et al.[43] prepared EGCG and Au nanoparticles (E-Au NPs) with good biosafety by one-step self-assembly in an aqueous solution, which can effectively prevent the formation of MRSA biofilm under near-infrared irradiation. Photothermal therapy (PTT) produces ROS, and the production of quinone protein is the characteristic antibacterial mechanism of polyphenols NPs (Fig. 3b). E-Au NPs with high-efficiency antibacterial properties and have no significant toxic side effects after treatment, which makes them promising candidates for potential clinical translation in antibiotic-resistant bacterial infections. MPN not only has a significant antibacterial effect but also has an inhibitory effect on harmful algae. Wang et al.[44] developed self-assembled nano-algaecides comprising black wattle TA and Cu2+ (CuBes). They found that the hydrophobic interaction, hydrogen bond, and π-π interaction between polyphenols and algal cell surface enable CuBes to attach to the surface of algae cells and achieve sustained targeted release of Cu2+. CuBes exhibited algal inhibition for more than 30 d (with an inhibition activity of ~99%) (Fig. 3c).
Figure 3.
(a) pH-dependent transition of FeIII-TA complex (MPN) state, and schematic representation of the transformation of CNC into CNC@MPN and the action of MPN occurring on the surface of CNC[41] ©2024, Wiley. (b) E-Au NPs were developed through direct one-step self-assembly methods, and the outstanding antibacterial effect involved in mild photothermal therapy, reactive oxygen species production, pathogenicity-related genes regulation, and quinoprotein formation[43]. (c) Preparation of CuBes and inhibition of algal cells[44] ©2023, ACS Publications. (d) Preparation of QFN and its anti-inflammatory and anti-senescent effects in protecting against ALF[48] ©2025, Ivyspring.
In other respects, the health benefits of MPN have also been well reported[45]. Copper ion-luteolin nanocomplexes (CuL NCs) were prepared as ROS scavengers to regulate the intestinal inflammatory microenvironment, CuL NCs reduced intestinal oxidative stress and inflammation by activating Nrf2 and inhibiting NF-κB, facilitating the transformation of macrophages to the anti-inflammatory M2 phenotype, thus increasing anti-inflammatory factors in the inflammatory microenvironment, and CuL NCs could protect intestinal cells from damage, and reduce pathogenic bacteria and enhance the richness and diversity of intestinal flora[46]. Different sources of plant polyphenols have diverse biological activities, and studies have shown that quercetin can act as a hepatoprotective agent[47]. A study by Feng et al.[48] showcased the synthesis of an ultra-small quercetin-FeIII nanoparticle (QFN, with sizes ranging from 2−3 nm). The simple coordination method of metal and polyphenols has the advantage of significantly improving the water solubility of quercetin. QFN was internalized by AML12 cells through membrane fusion (including various endocytosis), and more than 90% of it was taken up in 6 h, it provides an effective in vivo delivery strategy for efficient ROS scavenging by quercetin. QFN prevents acute liver failure (ALF) and disrupts the vicious cycle between inflammation and cellular senescence. (Fig. 3d).
-
Hydrogels are three-dimensional crosslinked natural or synthetic polymeric networks, an eminent category of soft materials[49], their water-rich nature has attracted significant attention in many areas, including tissue engineering, drug delivery, soft electronics, and biosensors[50−53]. Polyphenol-containing hydrogels can provide wet adhesion, ductility, and self-healing properties, they can simulate the rigidity and elasticity of genuine tissues, providing a moist environment for wound healing as a 'smart' soft material for biomedical applications[50,54,55]. Lian et al. developed a sprayable hydrogel dressing containing Mg-gallic acid metal-organic framework (Mg-GA MOF), in which the quaternary ammonium salt chitosan and released GA are effective antibacterial components (Fig. 4a), Mg-GA was synthesized by microwave-assisted synthesis with good dispersion, its simple and efficient synthesis method enables large-scale production. The Mg-GA MOF with enzyme catalytic activity can protect immune cells in an oxidizing environment, accelerate the transformation of M1-type to M2-type, and achieve long-term maintenance of oxidative stress. Hydrogel degradation is accompanied by the release of Mg2+, which promotes the reconstruction of the neurovascular network[56].
Figure 4.
(a) Sprayable hydrogel dressing containing Mg-GA MOF[56] ©2023, Elsevier. (b) ZPTA-G/HMA cross-links under GL irradiation to generate ROS to realize in situ cross-linking, adhesion, hemostasis and antimicrobial functions in one step[60] ©2023, Elsevier. (c) Synthesis of SalB-CuNCs nanozymes and preparation of the nanozyme-based hydrogel system, enzyme-like cascade reaction, oxygen-supplying and angiogenesis promotion effect of COC@SalB-Cu hydrogel[64] ©2024, Wiley. (d) Schematic diagram of the CETHR coating[67] ©2024, ACS Publications.
The crosslinking process of hydrogels transitions from a precursor solution to a gel. Thus, hydrogels can be used as injectable materials to deliver therapeutic bioactive molecules and subsequently gel within the target tissue[57]. Zhong et al. prepared an injectable self-healing hydrogel in which EGCG was incorporated into the hydrogel network, the self-healing performance is derived from the combined reversible diol-borate ester bonds and the interaction of hydrogen bonds. In addition, the pH-sensitive degradation behavior of the hydrogel allows EGCG to be released in a controlled manner during wound healing. Based on the fact that the catechol group can bind to the bacteria, an antibacterial strategy of 'capture and kill' was proposed, and the antibacterial rate of the hydrogel against S. aureus and E. coli was more than 96%[58]. Injection hydrogel can also be used as a biological adhesive, and in combination with the universal adhesiveness of TA, can be used to prepare hemostatic materials[22,59]. Liu et al. prepared the double network mucoadhesive ZPTA-G/HMA using modified gelatin as a gel matrix (G/HMA), polydopamine-modified zinc oxide (ZP) as a photocatalytic antibacterial substance and the polyphenol groups in TA provided anchorage. ZPTA-G/HMA has good adhesion and hemostatic properties. The adhesion strength of the hydrogel on gingival mucosa reached 46.25 ± 3.524 kPa. Under light irradiation, ZPTA-G/HMA can quickly cross-link to achieve hemostasis, and the release of TA combined with the photothermal effect of ZP reduces the secretion of inflammatory factors (Fig. 4b). Furthermore, ZPTA-G/HMA also has obvious advantages in terms of comfort and retention rate compared with commercial oral patches[60].
Although some hydrogels have been extensively studied for their biodegradability, hydrophilicity, and super absorbency, the main issues of insufficient adhesive force, and mechanical properties have yet to be solved[61]. The anti-adhesion of water severely limits the application of hydrogel adhesion to the surface of biological tissue. It has been reported that the adhesion properties of the catechol group make it well-adhered to various substrates[62]. For instance, the dynamic covalent bonding between EGCG and 3-acrylamido phenylboronic acid derivatives served as a stimuli-responsive cross-linker, directing the copolymerization of acrylamide via radical chain propagation. The elongation at break of the hydrogel was 661.3 ± 43.4%, and the maximum tensile strength was 29.0 ± 6.6 kPa. It can withstand the deformation of three times elongation, and the strong adhesion does not cause the formation of residues on the skin tissue[63]. The introduction of thymoquinone and natural photosensitive antioxidant curcumin into the hydrogel results in the formation of strong hydrogen bonds and π-π interactions with the amino containing (PEG-PAF). These forces bring the transition temperature of the gel (30 °C) close to the wound microenvironment, allowing the solution to be rapidly converted into a gel to cover the wound. Under blue light excitation, the ROS generated by the hydrogel can quickly be sterilized, and the curcumin endows the hydrogel with temperature-sensitive properties and effectively inhibits the expression of pro-inflammatory factors in cells. Polyphenols also have a proangiogenic effect. For example, the polyphenolic compound salvianolic acid B (SalB) was used to prepare self-cascade superoxide dismutase-peroxidase nanozymes (SalB-CuNCs) via polyphenol-copper coordination (Fig. 4c). The catechol group in SalB promotes the valence shift of copper ions and the trapping of superoxide anions, which endowed SalB-CuNCs with enzyme-like activity. Encapsulation of SalB-CuNCs in hydrogels improved the enzyme-like catalytic stability, and the hydrogels up-regulated the expression of vascular-related genes, significantly promoting angiogenesis under hypoxic conditions[64].
In addition, EGCG can reduce myocardial apoptosis and ameliorate myocardial oxidative loss[65,66]. Wan et al. prepared a complex of EGCG and tanshinone IIA sulfonic sodium (TSS) by π-π stacking interaction, and used the complex to construct a polyphenol-reinforced medicine/peptide glycocalyx-like coating (CETHR) with carboxylated chitosan and hyaluronic acid grafted with 3-aminophenylboronic acid (Fig. 4d). The components in the coating can modulate several signaling pathways associated with inflammatory response, cardiomyocyte apoptosis, and metabolism. CETHR coating can effectively inhibit platelet and whole blood adhesion, with good anti-thrombotic properties, suitable as a functional coating for heart blockers[67]. In summary, multiple forms of hydrogels protect polyphenols from external environmental damage, sustained release can be achieved, and the topically administered treatment modality reduces side effects.
-
Chitosan (CS) is a natural alkaline polysaccharide with good biocompatibility and antimicrobial properties. However, there are limitations to its poor mechanical properties and solubility, and it is usually combined with other materials to obtain superior composites used in applications including wound healing materials, bio-adhesives, and drug delivery systems[68,69]. A simple combination of the NH3+ group of CS grafted with the OH group of polyphenol extracted from grape pomace and then binding to hydroxyapatite formed a stable coating, the electrostatic complexation between polyphenols and CS governs controlled release kinetics in inflammatory microenvironments, while maintaining a residual polyphenol reservoir that sustains antioxidative efficacy through persistent ROS scavenging capacity[70]. In addition, the NH3+ group within CS gives it a positive charge, which helps to improve the stability of the emulsion system and acts as an emulsion stabilizer[71]. The preparation of CS-Laminaria japonica polyphenols (CP) and CS-Ascophyllum nodosum polyphenols (CB) into stable Pickering emulsions can be used as hydrophobic β-carotene delivery systems with antimicrobial capacity. The polyphenols in seaweed and CS are bound through hydrogen bonding and electrostatic interactions, forming an amorphous zone, which can be tightly bound at the oil-water interface. CS emulsions without polyphenols showed significant delamination after 1 week. In contrast, CP and CB Pickering emulsions showed few signs of delamination after 8 weeks, and both CP and CB were more resistant to heat treatment and UV irradiation. The β-carotene in the microemulsions demonstrated high bioavailability[72]. In addition, it has been reported that the photothermal stability of litchi polyphenols has increased after encapsulation by CS, and the material inhibited the growth of S. aureus and E. coli by releasing the polyphenols through cell penetration[73].
Due to the limitation of CS solubility, chemically modified CS derivatives have attracted much attention for their application in biomedical fields[69]. For example, in ethylene glycol CS-based hydrogels containing EGCG prepared using tyrosinase-mediated one-pot synthesis, EGCG acted as a cross-linking agent involved in bridging the CS hydrogel backbone, which was oxidized by tyrosinase and reacted with primary amines on CS in an oxidative reaction to form a hydrogel (Fig. 5a). As the concentration of EGCG increased, the pore size of the hydrogel decreased and the level of tumor necrosis factor-alpha (TNF-α) cytokine secretion was reduced, in addition, the hydrogel also reduced the inflammatory response of RAW 264.7 macrophages[74]. Additionally, quaternary chitosan (QCS), which contains a large number of cations, has strong tissue adhesion and was combined with TA, the presence of dynamic ionic and hydrogen bonds between QCS and TA, the injectable hydrogel has good self-healing and adhesion properties (Fig. 5b). Due to the good hemostatic activity of TA, coupled with the good adhesion of the hydrogel to the peri-wound tissue binding and plugging the bleeding site, it showed a wound healing rate of 92.8% after 14 d of treatment, which was higher than that of the commercial dressing Tegaderm[75].
Figure 5.
(a) Schematic illustration of the fabrication of EGCG CS hydrogel and the cross-linking mechanism of EGCG-CS hydrogel[74] ©2020, Elsevier. (b) QCS/TA hydrogels were injected into the site of injury[75] ©2022, ACS Publications. (c) Schematic illustration of the preparation of Cu-TA nanocomposite films[79] ©2025, Elsevier. (d) Schematic illustration of the construction of the functionalized CS hydrogel and its application in myocardial infarction repair[68] ©2024, Wiley.
The excellent film-forming properties of CS have made CS composites favored in antibacterial packaging materials[76]. The existing commonly used food packaging materials include plastics, paper products, and metals, all of which have the risk of contaminating food, thus, the development of degradable and multifunctional composite packaging materials has received great attention[77,78]. A previous study showed that Cu-TA nanoparticles doped into a QC-gelatin can be prepared as nanocomposite films, the film doubled the shelf life of strawberries and was more than 99% bactericidal against S. aureus and E. coli. In addition, the mechanical properties of the composite film were increased from 8.6 to 15 MPa, and the tensile strength was also increased by 1.75 times, indicating that the composite film is suitable for use as an excellent fruit preservation material[79] (Fig. 5c). Another investigation revealed that EGCG loaded melanin-like nanoparticles (EGCG@MNPs) incorporated into CS were used as nanocomposite films for active food packaging. The excellent antioxidant capacity of the nanocomposite film was attributed to the large number of phenolic hydroxyl groups in EGCG which can react with free radicals to prevent food oxidation[80,81].
Similar to TA, the abundant phenolic hydroxyl groups in proanthocyanidins (PAs) can also provide binding sites for metal ions. Li et al. prepared hydrogel patches based on the cross-linking effect of PA on CS and the chelating effect of PA on Eu3+, which were able to promote cardiomyocyte maturation and achieve adaptive repair of myocardial infarction (Fig. 5d). Besides, the hydrogel has a dual network structure, and its Young's modulus (45−50 kPa) matches natural myocardial tissue (20−500 kPa) for infarcted myocardium to provide long-term mechanical support. It has good biocompatibility and antioxidant properties and significantly improves contractile activity and wall thickness of the heart and inhibits cardiomyocyte fibrosis[68].
-
Other polyphenol-based biomaterials such as polyphenol eutectogels, polyphenol polymers, and coating film materials have also been developed for antimicrobial or drug delivery systems[82,83]. Phenolic hydroxyl groups in natural polyphenols have been shown to act as hydrogen bond donors in synthesizing new green solvents deep eutectic solvents (DES)[84]. Novel polymerisable DES (PDES) prepared by TA, choline chloride, and hydroxyethyl methacrylate by hydrogen bonding and van der Waals forces. PDES with low viscosity and high C = C conversion can be directly 3D printed to arbitrary shapes with antimicrobial and UV-resistant materials[85]. Moreover, DES synthesized from a variety of natural polyphenols with quaternary ammonium salts formed low-cohesive eutectogels combined with amino methacrylic acid, where strong interactions between the monomers dramatically lowered the melting point of the mixture, and the eutectogels can be stretched by more than 1000%, as well as possessing antimicrobial properties and metal complexing capabilities[84]. Similarly, therapeutic DES (THEDES), synthesized from choline and geranic acid, have strong skin penetration and drug solubilization properties, allowing them to be used as new dermal drug delivery vehicles, and the liquid form avoids using additional solvents. The eutectogels prepared by simply heating and mixing THEDES with gelatin and TA exhibited similar skin penetration enhancement capabilities and were also very biocompatible (Fig. 6a). This new soft material provides good ideas for the application of therapeutic soft materials in the fields of medicine and biology[83].
Figure 6.
(a) Fabrication process of eutectogels[83] ©2024, Wiley. (b) Schematic diagram of the formation of coating through polyphenolic polymers on the surface of tumor cells for metabolite inhibition[86] ©2024, Elsevier. (c) Representative drug delivery systems for polyphenol[21] ©2023, MDPI. (d) Schematic illustration of EGCG-ACP promotion of enamel remineralization and prevention of demineralization in vitro, and its restoration of enamel remineralization in the presence of bacteria in vivo[91] ©2023, Elsevier.
A variety of polyphenols have been demonstrated that can bind to polymers. For example, polyphenol polymers (PPA, PGA, PGTP, PTA) were prepared by combining protocatechuic acid (PA), GA, tea polyphenol (GTP), and TA as polyphenol group donors with polylysine (PL), respectively. They can significantly agglomerate and adhere to the surface of tumor cell membranes upon oxidation to form a dense, impenetrable polymer network. Polyphenolic polymers can adhere to the tumor site for extended periods, to inhibit the uptake of nutrients such as glucose and reduce the production of lactic acid, GSH, ATP, and induce the production of a large number of ROS to inhibit the growth of tumor cells (Fig. 6b)[86]. In a similar study, Yi et al.[87] constructed a library of polyphenol amino acid colloidal sphere materials using EGCG with different amino acids, and efficiently prepared nanomedicines by microfluidic technology, which did not aggregate even at higher concentrations, facilitating clinical application. EGCG polymer colloid spheres significantly improved the drug loading and intracellular uptake of insoluble drugs, they showed good anti-tumor effects in vivo experiments without significant side effects. Commonly used TA also has various roles in the synthesis of antitumor nano drugs. The hydrogen ions released by TA play an etching role for ZIF-8, and then TA binds to proteins to form polyphenolic protein polymers, ultimately synthesizing hollow nanosphere of metal-protein-polyphenolic materials. TA replaces strong acids and better preserves the enzyme activity for anti-tumor efficacy[88].
The combination of polyphenols with other substrates can render good antimicrobial, antioxidant, and mechanical properties of the material[55]. For example, TPs were ester-exchanged with polylactic acid and doped into polystyrene-methacrylic acid-triglyceride, the composite film showed 99.99% inhibition of S. aureus and E. coli, which is expected to be used as an antimicrobial food packaging material[89]. Synthesis of EGCG-poly(acrylic acid) conjugates solved the problem of rapid autoxidation of EGCG, and the oxidative stability and cytocompatibility of these nanoparticles were superior to that of monomeric EGCG. In addition, the surfactant function of poly(acrylic acid) improved the stability of the nanoparticles and gave the conjugates a micellar behavior[90]. For some polyphenols with low water solubility, it has been reported that combining them with liposomes, phospholipid complexes, polymer ions, micellar materials, and dendrimers can improve the solubility, stability, and biocompatibility of polyphenols (Fig. 6c)[21]. Regarding other substrate materials, a recent study reported that a functional nanocomposite EGCG-ACP was synthesized by chelating EGCG and amorphous calcium phosphate (ACP)[91]. EGCG-ACP exhibited favorable biocompatibility and can promote the protonation of EGCG to play an antimicrobial role in acidic conditions, and also protect the enamel. Besides, the high release of ACP in the presence of cariogenic bacteria slows down the process of demineralization, prevents dental caries, and promotes remineralization of enamel. EGCG-ACP overcomes the difficulty of restoring demineralized enamel in a cariogenic acidic environment (Fig. 6d).
-
This review summarizes the research progress on biomaterials constructed by co-assembling plant polyphenols with various substances. These polyphenolic biomaterials exhibit excellent stability and biological activity, with the self-assembly of polyphenol nanoparticles and green-synthesized MPNs demonstrating good biocompatibility along with significant antioxidant and antimicrobial capabilities. Polyphenol-based soft materials for medical and healthcare applications can be utilized as wound dressings and may also function as heart blockers. Although the types and applications of biomaterials derived from plant polyphenols are increasing and have shown strong capabilities across various research areas, the translational aspects of these materials in clinical settings remain in their infancy, and there are currently inadequate procedures for assessing their toxicology and safety. In conclusion, these strategies for synthesizing polyphenol biomaterials provide valuable references for functional applications of plant polyphenols. We believe that this review will offer systematic information to researchers and stimulate more innovative research on plant polyphenols, thereby accelerating their practical application in biomaterials.
This study was supported by the National Natural Science Foundation of China (32372757, 22174129), Sichuan Provincial Key Research and Development Program (2024YFHZ0179), the Innovative Program of Chinese Academy of Agricultural Sciences (CAAS-ASTIP-2021-TRI), the Agriculture Research System of China of MOF and MARA (CARS-19), and the Natural Science Foundation of Zhejiang Province (LZY21E030001).
-
The authors confirm contribution to the paper as follows: writing-original draft preparation: Wang S; writing-review and editing: Wang S, Wang Z; Zhang X; investigation: Wang S; Wang Z; supervision: Li Z; Zhang X; Chen H; validation: Li Z; Zhang X; Chen H. All authors reviewed the results and approved the final version of the manuscript.
-
Data availability is not applicable to this article as no new date were created or analyzed in this study.
-
The authors declare that they have no conflict of interest.
- Copyright: © 2025 by the author(s). Published by Maximum Academic Press, Fayetteville, GA. This article is an open access article distributed under Creative Commons Attribution License (CC BY 4.0), visit https://creativecommons.org/licenses/by/4.0/.
-
About this article
Cite this article
Wang S, Wang Z, Li Z, Zhang X, Chen H. 2025. Recent advances in tea and other plant polyphenol biomaterials for antibacterial and disease treatment. Beverage Plant Research 5: e010 doi: 10.48130/bpr-0025-0012
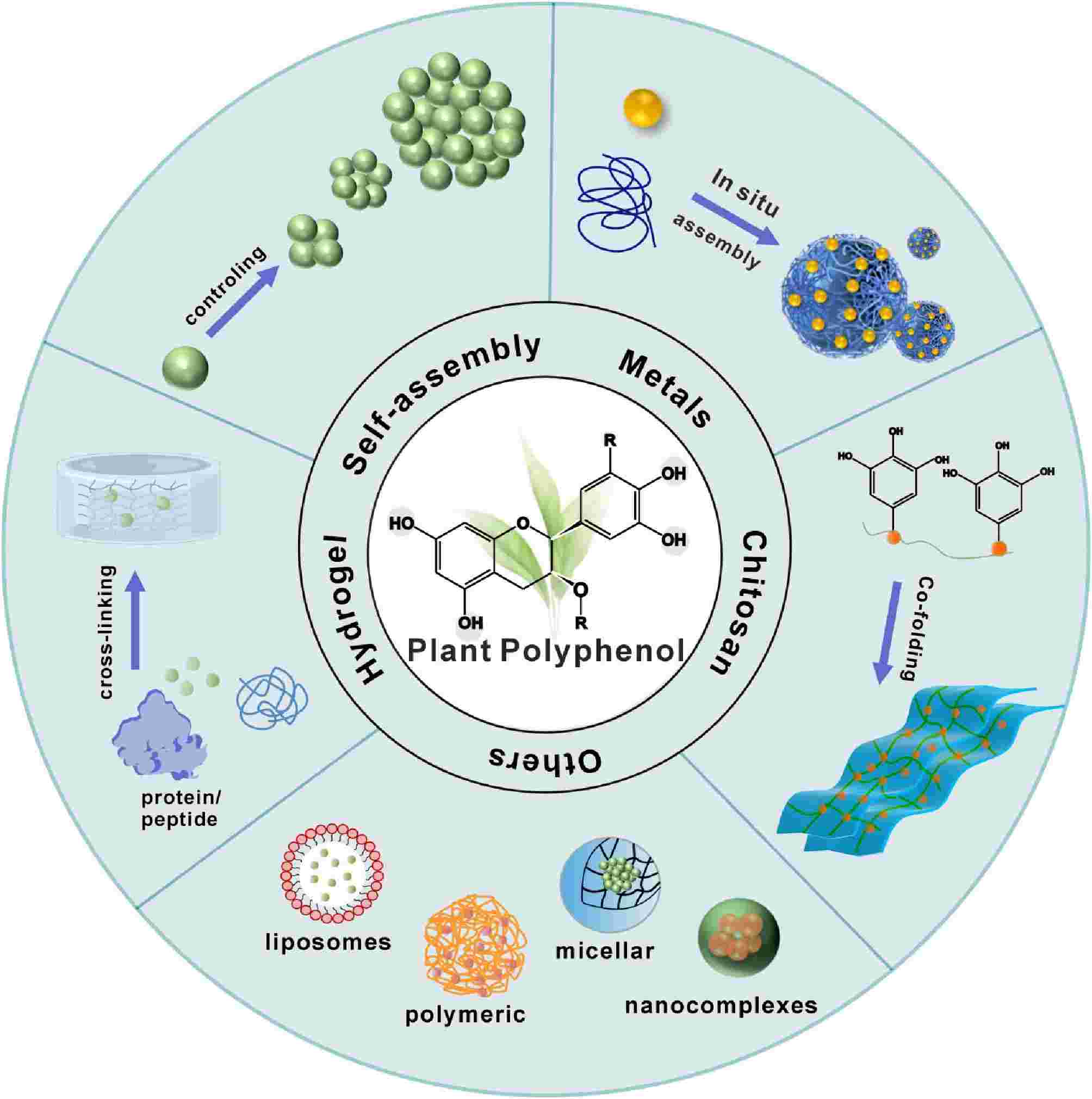