-
The microbial community in the gut significantly impacts various physiological processes, including food metabolism, vitamin synthesis, gut mucosal barrier integrity, immunomodulation, and pathogen defense[1]. Disruptions in the gut microbiota are associated with various metabolic diseases, such as obesity, diabetes, anxiety, and depression[2,3]. Microbial metabolites are synthesized de novo by bacteria or formed through bioconversion of foreign substances (mainly dietary nutrients) or host-derived compounds[4]. Emerging evidence highlights the involvement of gut microbiota in host physiology and metabolic pathways, largely through the production of diverse metabolites[5]. Consequently, these gut microbiota-associated metabolites are pivotal in unraveling the mechanisms that govern host health.
A significant challenge in studying the health effects of microbes is differentiating metabolites produced by the microbiota and those generated in human tissues or directly derived from dietary sources. Metagenomic analysis, which catalogs the genes present in the microbial environment, aids in providing mechanistic insights into the metabolic changes linked to disease[6]. Metabolomic analysis explores which compounds may mediate the relationship between microbial activity and host disease, offering the possibility of interpreting various metabolite sources[7]. The gut microbiota can convert certain compounds into active metabolites with improved bioavailability and therapeutic properties than their original forms[8−10]. Representative compounds transformed by the gut microbiota include alkaloids, flavonoids, and terpenoids[11,12]. These naturally occurring active ingredients are widely present in various foods and, even at low levels, can significantly contribute to anti-inflammatory, antioxidant, and lipid-lowering effects, which can help improve metabolic diseases[13]. Further clarification of these novel metabolite types and their action mechanisms is crucial for understanding how the gut microbiota mediates dietary bioactivity.
Dietary nutrients, frequently altered during food processing, are a primary factor influencing gut microbial-related metabolites. Throughout processing, dietary nutrients undergo various changes, affecting microbial metabolism in different ways[14]. This review summarizes three distinct sources of microbial metabolites and describes the regulatory roles of representative microbial metabolites from each category in host disease states. Moreover, it details the roles of three novel bacterial metabolites—alkaloids, terpenoids, and flavonoids—in specific aspects of human health, as well as their interactions with the gut microbiota. The aim is to clarify the mechanisms involved and enhance understanding of how specific bacterial species and metabolites influence human health. Additionally, how food processing influences the nutritional makeup of the diet and its potential effects on disease management are highlighted, which is essential for assessing the relationship between diet and health.
-
Metabolites involved in gut microbiota are crucial mediators of their efficacies[15]. These metabolites can broadly be classified into three categories: (i) those synthesized de novo by gut microbes; (ii) those produced by gut microbes directly from dietary components; and (iii) those produced by the host and subsequently modified by gut microbes[13]. Some of these metabolites may have multiple sources, and here representative examples of these metabolites in most cases are summarized and discussed (Fig. 1).
Synthesized de novo by gut microbes
-
Research indicates that the gut microbiota can synthesize vitamin K and the majority of water-soluble B vitamins like biotin, riboflavin, thiamine, folates, pantothenic acid, and nicotinic acid[16]. For instance, cobalamin (vitamin B12) is exclusively produced by microbes, specifically anaerobic bacteria[17]. Genomic analysis combined with in vivo and in vitro metabolic experiments confirmed that Cetobacterium somerae CS2105-BJ is capable of de novo vitamin B12 synthesis. It contains essential genes (hemL, cbiT/cobD, and cobC) that play a role in various stages of B12 biosynthesis, which are responsible for the synthesis of uroporphyrinogen III, adenosylmethylcobalamin, and lower ligand, respectively[18,19]. In situations where dietary vitamin B2 was depleted in a mouse model, the gut microbiota provided short-term compensation[20]. Certain vitamins, like B vitamins, which serve as precursors to essential metabolic cofactors, can be synthesized by specific gut bacteria known as prototrophic bacteria. However, they must also be obtained from other bacterial species (auxotrophic bacteria) and from the host’s diet[21]. This highlights that microbial synthesis of specific metabolites may depend on environmental conditions and the availability of exogenous sources, necessitating multi-bacterial cooperation. Additionally, amino acids and fatty acids can be synthesized de novo by gut microbes. Research has demonstrated ruminal bacteria such as Selenomonas ruminantium, Streptococcus bovis, and Prevotella bryantii engage in amino acid synthesis under physiological conditions, particularly enriching glutamic acid and aspartate in the presence of peptides[22]. Bacteroidetes are known symbionts capable of sphingolipid production. For example, Bacteroides thetaiotaomicron can initiate biological sphingolipid synthesis via serine palmitoyl transferase[23].
Metabolism of dietary components by gut microbes
-
Microbial dietary metabolites include short-chain fatty acids (SCFAs), trimethylamine oxide (TMAO), and indole and its derivatives. SCFAs, primarily derived from dietary fiber fermentation, are a major focus. For example, resistant starch type 3 from potatoes promotes the production of propionic and acetic acids by Bifidobacterium, Ruminococcus, Bacteroides, and Coprococcus[24]. Pectin, rich in rhamnogalacturonan-I, significantly promotes the proliferation of Bifidobacterium, Faecalibaculum, and Lactobacilli in C57BL/6J mice, and it is metabolized to SCFAs[25]. Dietary inulin supports SCFA-producing Ruminococcaceae and Lachnospiraceae, increasing fecal SCFA concentrations[26]. Specifically, Bifidobacterium produce lactate and acetate, Bacteroides generate propionate and acetate, and Butyrivibrio and Fusobacterium produce butyrate as their primary metabolites[27]. Other bacteria that produce SCFAs include Anaerostipes, Anaerotruncus, Bacteroides, Coprococcus, Clostridium, Dialister, Eubacterium, Faecalibacterium, Lactobacillus paracasei, Odoribacter, Parabacteroides, and Ruminococcus[28−32].
Tryptophan metabolites, particularly indoles, such as 3-indolepropionic acid, indoleacetic acid, indole acrylate, indoleacrylic acid, indole-3-carbaldehyde, and tryptamine, form another extensively studied group of dietary metabolites[33]. For instance, 3-indolepropionic acid is biosynthesized from tryptophan by Clostridium sporogenes[34]. Commensal Peptostreptococcus metabolizes tryptophan into indoleacrylic acid[35], while Bifidobacterium longum CCFM1029 produces indole-3-carbaldehyde[36]. Putrescine, derived from arginine through a hybrid pathway involving Escherichia coli and Enterococcus faecalis, is another notable metabolite. Bifidobacterium spp. that produce acidic compounds accelerate putrescine production[37].
Choline metabolites are classic nitrogenous dietary compounds. The synthesis of TMAO increases following the consumption of a diet high in phosphatidylcholine and L-carnitine[38]. Gut microbiota, like Citrobacter freundii, are capable of transforming into trimethylamine (TMA), which is then oxidized to TMAO[9]. Research has indicated that trigonelline can reduce TMAO levels by inhibiting the activity of C. freundii and flavin-containing monooxygenase 3 in mice. In vitro experiments also showed that the addition of fenuelline to choline-rich medium could significantly inhibit the production of C. freundii and reduce TMA and MAO production[9,39]. In another study involving obese hypertensive individuals, Lactobacillus plantarum was found to decarboxylate ornithine to produce putrescine[40]. Spermidine production is facilitated by some gut microbiota, such as E. coli and Bacteroides, utilizing carboxyspermidine decarboxylase and spermidine synthase[41,42]. Additionally, inosine, as a purine nucleotide metabolite, may be produced by Lactobacillus during the fermentation of barley leaves[43]. In general, there are a wide variety of microbial metabolites from dietary sources, and the efficacy of many substances, including SCFAs and indole derivatives, have been extensively studied with their relevant mechanism identified. Whether new dietary metabolites with good efficacy beyond these substances can be identified needs further exploration.
Host metabolism followed with gut microbe modifications
-
The liver serves as the primary organ to regulate cholesterol homeostasis, controlling both cholesterol intake and de novo synthesis. Endogenous cholesterol synthesis is mainly regulated by the SCAP-SREBP-HMGCR pathway[44,45]. In addition, a microbial enzyme called ismA has been identified previously that can convert cholesterol into coprostanol, a lipid that is excreted from the body rather than being absorbed[46]. Yao et al.[47]identified a gene encoding the sulfotransferase (BtSULT, BT0416), which is widely found in Bacteroides and mediates the sulfation of cholesterol.
Bile acids (BAs), which are synthesized in the liver from cholesterol under the action of cholesterol- 7α-hydroxylase (CYP7A1), and are conjugated with taurine or glycine, undergo modifications by gut microbiota to produce secondary BA metabolites[43,48,49]. Pruss et al.[50] used stable isotope tracers along with bacterial and host genes to uncover a common metabolic pathway of hippuric acid production by host microorganisms. They confirmed that Clostridium sporogenes reduced phenylalanine into phenylpropionic acid[50-51]. The third type of metabolite, which is metabolized by the host and subsequently modified by microorganisms, has not been detailedly explained in most studies. This complexity likely arises from the intricate interactions between the host and gut microbiota, which makes it challenging to distinctly delineate their respective roles in metabolite modification.
-
Metabolic diseases, such as diabetes, atherosclerosis, hypertension, and obesity, are often linked with dysregulation of gut microbial metabolites[9]. Natural products-derived metabolites, including alkaloids, terpenoids, and flavonoids, undergo substantial modification by gut microbiota, affecting their pharmacological effects and roles in metabolic diseases[52]. These compounds typically exhibit low absorption rates in intestinum tenue and are further broken down by microbiota in the colon into microbial metabolites, which have been confirmed to play a significant role in regulating metabolic diseases[52]. The following section will specifically explore the interaction between these diet-derived metabolites and gut microbiota, as well as their functions in metabolic diseases (Fig. 2).
Figure 2.
Natural product-derived gut microbial metabolites in metabolic diseases. Different colors refer to different classes of metabolites, with red representing alkaloids, green representing terpenoids, and yellow representing flavonoids. The blue boxes indicate key sites on the pathways, and the brown boxes indicate key sites shared by the three metabolites (created with BioRender.com).
Alkaloids
-
Gut microbiota are essential for the absorption of alkaloids, such as Staphylococcus aureus, Enterococcus faecalis, and Enterococcus faecium, and they possess nitrate reductase enzymes that metabolize alkaloids[53,54]. Previous studies have indicated that the cytochrome CYP450 family, including CYP2D6, CYP1A2, CYP51, and CYP7A1, plays a significant role in the metabolic transformation of alkaloids[55−57], including O-demethylation and hydroxylation[55 ]. Alkaloids can influence the body’s ecological physiology by regulating gut microbiota and their metabolic products. Supplementation with alkaloids increases the abundance of Bacteroides, Parabacteroides[58], Ruminococcus[59], Blautia[60], Faecalibaculum, Allobaculum[61], Clostridiales, and Lactobacillus[62], which are shown to produce SCFAs, TMAO, and BAs[61,63,64].
The primary causes of obesity are abnormalities in energy balance and weight regulation, often accompanied by enlarged fat cells and changes in insulin function, which can further lead to the development of diabetes. Peroxisome proliferator activated receptor alpha (PPARα) and peroxlsome proliferator-activated receptor-gamma coactivator-1 alpha (PGC1α) are associated with the proliferation of adipose tissue and adipocyte hypertrophy following dietary intake, while targets such as liver X receptor (LXR), farnesoid X receptor (FXR), and CYP7A1 play crucial roles in regulating lipid, glucose, and energy metabolism, making them key targets for the treatment of metabolic diseases such as obesity and diabetes[65−67]. Alkaloids can effectively act on the above targets and play a key role in disease improvement. For instance, palmatine treatment can regulate BA metabolism, reduce cholesterol accumulation, and alleviate obesity by enhancing the expression of PPARα and CYP7A1 while suppressing the expression of FXR[68]. Berberine can inhibit hepatic lipogenesis by up-regulating the expression of intestinal FXR and fibroblast growth factor 15, as well as activating the AMP-activated protein kinase (AMPK)-dependent Raf-1 pathway, thereby affecting the expression of the low-density lipoprotein receptor (LDLR)[62,69]. Notably, LDLR is a transmembrane glycoprotein primarily responsible for clearing lipoproteins, and an effective therapeutic target for treating hypercholesterolemia and related cardiovascular diseases[69]. In diabetic mice, supplementing with ruthenine improved the disease by activating the PPARα/PGC1α signaling pathway and upregulating β-oxidation-related genes in the liver[70]. In addition, studies have shown that Streptococcus faecalis can convert berberine in Rhizoma coptidis into oxyberberine, a potent hypoglycemic metabolite[54]. This metabolite contributes to anti-diabetes by upregulating the mRNA expression of the pancreatic nuclear factor erythroid 2-related factor 2 (Nrf2) signaling pathway and activating the phosphatidylinositol 3-kinase (PI3K)/protein kinase B (Akt) signaling pathway[71].
Alkaloids exhibit anti-inflammatory properties by inhibiting pathways such as TRIF-dependent NF-κB signaling. For example, oxyberberine modulates the toll-like receptor 4 (TLR4)-myeloid differentiation factor 88 (MyD88)-NF-κB pathway[54] and the TLR4/PI3K/NF-κB pathways, reducing inflammatory responses[71]. For another, berberine can exert its effects by inhibiting the phosphorylation of insulin receptor substrate-1 (IRS-1) and increasing the phosphorylation of AKT in adipose tissue and cultured adipocytes[72]. These studies highlight the significant role of alkaloids in human health by interacting with gut microbiota and their metabolic products, thereby contributing to the maintenance of homeostasis. Despite extensive research on the structure and physiological activity of alkaloids metabolites, investigations into their in vivo roles and specific mechanisms in regulating human health are still limited.
Terpenoids
-
Terpenoids have demonstrated efficacy in ameliorating metabolic disorders by regulating gut microbiota. Studies indicate that terpenoids such as fucoxanthin affect the Firmicutes/Bacteroidetes ratio and influence the abundance of key species, which are pivotal in anti-obesity activities[73]. Additionally, terpenoids have been shown to enhance populations of Lactobacillus, Bifidobacterium, Clostridium sensu stricto 1, Turicibacter, uncultured Erysipelotrichaceae, Faecalibaculum, and Ruminococcus, while reducing Lachnospiraceae NK4A136, uncultured Lachnospiraceae, and taxa such as Prevotellaceae UCG-003 and Oscillospira[74−76]. In another context, the administration of ginsenoside extract enriched gut E. faecalis significantly[77]. Zhang et al.[78] confirmed that a guava extract abundant in 1-3 glucose-based residues of triterpenoid glycosides has significantly regulated Elusimicrobium, Lachnospiraceae UCG-004, acetic acid, butyric acid, and 1β-hydroxycholic acid in T2DM rats. Gentiopicroside has been shown to directly bind FGFR1, promoting FGF21 signaling, and subsequently activating PI3K/AKT and AMPK pathways to regulate glucose and lipid metabolism[79]. Reports indicate that gentiopicroside can be metabolized by intestinal bacteria into potential pharmacologically active metabolites such as erythrocentaurin, gentianine, and gentianal[80]. Consumption of erythrocentaurin can inhibit α-amylase activity, improving postprandial hyperglycemia, while supplementation with gentiopicroside may reduce inflammation by inhibiting TLR4/NLRP3-mediated pyroptosis[80,81].
The anti-inflammatory mechanisms of terpenoids involve several key factors. Glycyrrhetic acid is a triterpenoid saponin extracted from licorice. It can be hydrolyzed by β-D glucuronidase of Eubacterium sp. strain GLH and then converted to Glycyrrhetic acid 3-O-mono-D-glucuronide, enhancing anti-inflammatory activity[82]. Han et al.[75]illustrated that lactucin curtails p38, extracellular signal-regulated kinase (ERK), and AKT phosphorylation within lipopolysaccharides (LPS)-stimulated RAW264.7 cells, consequently diminishing the mRNA and protein levels of inducible nitric oxide synthase and cyclooxygenase-2, and attenuating interleukin-6 (IL-6) production. Jolkinolide B mitigates LPS-induced degradation of NF-κB inhibitor alpha (IκBα) and phosphorylation of p65 and MAPK, subsequently reducing histological alterations and inflammatory markers[83]. Limonin, a triterpenoid extracted from citrus, mitigates intestinal inflammation by downregulating p-STAT3/miR-214 levels, demonstrating anti-inflammatory and apoptotic effects[84]. Fucoxanthin, derived from marine algae, activates Nrf2/Antioxidant response element (ARE) through PI3K/Akt pathways, enhancing antioxidant synthesis[85−87]. Fucoxanthin also activates AMPK, reducing fatty acid synthesis via the sirtuin 1 (SIRT1)/AMPK pathway[88]and improving mitochondrial function while reducing oxidative stress in endothelial cells through the AMPK-Akt-cAMP response element-binding protein (CREB)-PGC1α pathway[89].
Phytol, a plastid terpenoid synthesized via the methylerythritol phosphate pathway in plastids, is released when chlorophyll molecules are partially digested in animals[90]. It is absorbed in the small intestine and converted to phytanic acid in the liver[91]. Phytanic acid activates PPARγ and the retinoid-X-receptor (RXR), regulating lipid metabolism in various cell types[92]. The activation of PPARγ in adipose tissues improves insulin resistance, while PPARα activation in the liver lowers circulating lipid levels[93,94]. Terpenoids derived from Resina commiphora modulate lipid metabolism by augmenting PPARα and carnitine palmitoyl transferase 1 (CPT1) expression[95]. Auraptene, a novel PPARα and PPARγ ligand found in citrus fruits exerts anti-atherosclerotic and anti-diabetic effects by balancing anti-inflammatory/pro-inflammatory cytokine levels in adipocytes[96]. These functions of dietary terpenoids are important for the control of metabolic diseases through dietary intervention.
Flavonoids
-
Flavonoids can be classified into several categories: flavones (including apigenin and luteolin), flavonols (including quercetin and curcumin), flavanones (including naringenin and hesperidin), flavanols (including catechin and epicatechin), isoflavones (including daidzein and genistein), and anthocyanins (including pelargonidin and cyanidin). They are primarily derived from fruits, vegetables, legumes, onions, seeds, plant roots and stems, tea, wine, olive oil, tomato sauce, and various dietary supplements[97,98]. After supplementing the diet with flavonoid-rich foods, such as soybean, bilberry, citrus fruit, and green tea, the enzymes produced by gut bacteria undergo various reactions including deglycosylation, demethylation, and oxidation, which generate biologically active metabolites with health benefits such as genistein, quercetin, anthocyanin, and catechin[99]. Santangelo et al.[100] summarized multiple studies showing that quercetin inhibits some intestinal bacteria such as Streptococcus, Lactobacillus, Bifidobacterium, and Bacteroides, and was converted into small molecules like phenylacetic acid, phenylpropanoic acid, dihydroxyphenylacetic acid, hydroxybenzoic acid, dihydroxybenzoic acid, and propionic acid, absorbed and utilized by the body[101]. The catabolism of cyanidin-3-O-glucoside in the gastrointestinal tract yields bioactive metabolites like protocatechuic acid, phloroglucinaldehyde, vanillic acid, and ferulic acid, supporting the integrity of the mucosal barrier and improving the health of the microbiota[102,103]. Escherichia fergusonii and E. coli metabolize curcumin into dihydrocurcumin, tetrahydrocurcumin, and ferulic acid[104]. In particular, Bifidobacterium animalis subsp. lactis AD011 efficiently converted 85% of quercetin 3-glucoside and isorhamnetin 3-glucoside into quercetin and isorhamnetin, respectively, within just 2 h without degrading the flavonoid backbone[105].
Flavonoids exert significant effects on metabolic diseases through interactions with key targets such as PPAR, AMPK, Nrf2, and NF-κB. For instance, they can bind to non-phosphorylated signal transducer and activator of transcription 3 (STAT3) in visceral adipose tissue, reducing its phosphorylation and transcriptional activity. This action leads to a decreased expression of the STAT3 target gene CD36, which typically influences adipogenesis and anti-visceral obesity by suppressing PPAR-γ expression[106]. Daidzein influences cardiac energy metabolism by modulating Sirtuin 3 (SIRT3), thereby improving lipid, glucose and ketone body metabolism disorders, and mitigating mitochondrial dysfunction, which is crucial for meeting cardiac ATP demands both in vivo and in vitro[107]. Daidzein influences the LXR signaling pathway, activating RXR, PPARα, and AMPKα, which enhances energy metabolism[108,109]. In skeletal muscle, daidzein inhibits the Glut4/AMPK/Forkhead box O (FoxO) pathway and reduces the expression of atrogin1 and muscle ring finger protein1, thereby inhibiting the protein degradation of the skeletal muscle[110].
Insulin induces GLUT-4 transport from the vesicle to the plasma membrane, thereby promoting glucose uptake in cells, through the PI3K/Akt pathway. Disruption of these pathways may lead to insulin resistance, which can subsequently result in diabetes[111]. Fraction D, rich in flavonoids isolated from Enicostema littorale, enhances cell glucose uptake by upregulating IRS-1/PI3K/Akt pathway components[112]. Both cyanidin-3-O-β-glucoside and pinocembrin inhibit inflammatory pathways like NF-κB and TLR4/MD2, providing therapeutic benefits for liver and intestinal inflammation[113,114]. Cyanidin-3-O-β-glucoside treatment stimulates SIRT1 activity, inhibits NF-κB acetylation, and subsequently inhibits the activation of inflammasomes and the release of pro-inflammatory cytokines in liver cell lines[113]. Quercetin in diet improves experimental colitis by enhancing the anti-inflammatory and antibacterial capacities of macrophages through the Nrf2/Heme Oxygenase 1 (HO-1) pathway[115]. Additionally, the inclusion of Eucommia ulmoides flavones leads to increased levels of phosphorylated Akt, IκBα, and IKKα/β, while simultaneously reducing the expression of Bax and Caspase-3 proteins in LPS-treated cells[116]. Other flavonoid metabolites, including protocatechuic acid, phloroglucinol, and vanillic acid, can downregulate the MAPK pathway by inhibiting the phosphorylation of ERK, c-Jun N-terminal kinase (JNK), and p38[117,118]. Overall, flavonoid metabolites play active roles in modulating various pathways, including NF-κB, MAPK, ERK, JNK, and p38, contributing to their anti-inflammatory and metabolic regulatory effects in different physiological conditions.
-
Food processing, including thermal processing and non-thermal processing, plays a crucial role in our daily diet[119]. These processes can alter the nutritional composition of food by introducing new compounds or converting existing ones[120] (Fig. 3). Considering that utilizing dietary interventions to improve metabolic diseases has become an effective means[121], its efficacy is highly correlated with gut microbiota metabolites as described previously. Therefore, comprehending the effects of food processing technology is crucial for assessing the nutritional value of processed foods and selecting appropriate processed foods based on differential health needs.
Figure 3.
Impact of food processing on dietary nutrients and their health benefits via gut microbiota and related metabolites. The blue arrows represent the impact of thermal processing, while the purple arrows represent the impact of non-thermal processing (created with BioRender.com).
Thermal processing
-
Heat treatments, such as boiling, baking, stewing, steaming, frying, and roasting, are widely used in both domestic and industrial food processing[122]. Previous research has indicated that the carbohydrate levels in grains, vegetables, and fruits typically rise following heat treatment[123, 124]. For example, heat-treated highland barley has been found to have a high dietary fiber content[124]. Similarly, it is found that boiling increases the content of fiber by 8.32% in garden cress seeds[125]. The increase in dietary fiber after thermal processing yields some beneficial outcomes, such as boosting the prevalence of beneficial gut bacteria including Bifidobacteria and Bacteroides, while suppressing harmful bacteria like Helicobacter and Enterococcus[126]. It also enhances the levels of SCFAs. These typical gut bacteria and metabolites are strongly associated with lipid metabolism and can effectively improve metabolic diseases[127,128]. The variability of these nutrients highlights that different processing methods may impact the efficacy of food intervention in varying ways, possibly due to changes in active components and their related metabolites[129,130].
Nevertheless, it is crucial to recognize that most nutrients, including proteins, phenols, vitamins, and minerals, are lost after thermal processing[123]. During heat treatment, protein structures can undergo modifications due to direct oxidation or complex reactions with other components of the food, leading to changes in processing characteristics and a reduction in nutritional value, with potential negative health implications[131]. For example, heat treatment of hawthorn has been shown to cause the degradation of soluble phenols and proanthocyanidins[132], which are vital in the gastrointestinal tract due to their antioxidant, anti-inflammatory, and antibacterial properties[133]. These compounds also promote the growth of probiotics like Bifidobacterium and Lactobacillus, offering potential health benefits. Additionally, steaming fresh tea significantly reduces the levels of vitamins (B2, B3, and C) and minerals[123]. Vitamin B2 has been reported to prevent cancer, hyperglycemia, hypertension, diabetes, oxidative stress, and other health conditions, while vitamin C is known for its anti-inflammatory, antioxidant, and hypoglycemic effects[134,135]. The loss of these nutrients significantly reduces the health benefits of processed foods. Taken together, while heat treatment can increase dietary fiber, it can also have varying degrees of negative effects on the original nutrients and health benefits of the diet.
Non-thermal processing
-
Non-thermal processing technologies, such as cold plasma treatment, irradiation, high-pressure processing, ultrasound, pulsed light technology, pulsed electric field treatment, and microbial fermentation, are widely used in food processing to circumvent the negative effects of heat[136]. These methods are designed to eliminate the need for high temperatures, thereby preserving the nutritional integrity of food[137]. For example, high-pressure electric field treatment has been demonstrated to elevate the anthocyanin content in strawberries, thereby improving its bioavailability[138,139]. In addition, ultrasound and ozone treatments have been found to increase the levels of phenols and vitamin C, and the antioxidant capacity of guava juice[140]. Mehta et al. found that cold plasma treatment for 10 min retained up to 95% of vitamin C [141]. These polyphenols and vitamins are crucial for human health[142], highlighting the potential of non-thermal processing to optimize the nutritional retention and even enhance the nutritional value of food products. As demonstrated by Tan et al.[143], high hydrostatic pressure treatment at 400 MPa for 15 min altered the monosaccharide composition of Cyanidin 3-glucoside and blueberry pectin complexes, decreasing mannose, fructose, glucose, xylose, and arabinose while increasing galactose, which improved colitis in mice through reducing intestinal oxidative stress, enhancing anti-inflammatory factors, and inhibiting the NF-κB signaling pathway mediated by the gut microbiota. Similar benefits have been observed with plasma treatment of orange juice and apple juice[144]. In terms of proteins, cold plasma treatment can oxidize hairtail muscle proteins, creating a more intensive protein network, which improves the texture and taste of the hairtail[145].
Microbial fermentation in food processing can lead to the formation of specific nutritional components due to the involvement of microorganisms. For instance, probiotic fermentation significantly increases γ-aminobutyric acid, rutin, total polyphenols, and total flavonoids in rice buckwheat[146]. Fermented rice buckwheat has been shown to reverse high-fat diet-induced intestinal dysbiosis, suppress hepatic cholesterol synthesis and lipogenesis, and stimulate lipolysis by rebalancing the Firmicutes/Bacteroidetes ratio, enhancing SCFA-producing bacteria like Bacteroidetes, Lactobacillus, and Blautia, and increasing total SCFA content[147]. Furthermore, blueberry residue fermented by Lactobacillus rhamnosus GG and Lactobacillus plantarum-1 significantly suppressed inflammation-related cytokines (tumor necrosis factor-α, IL-1β, IL-6), upregulated PPAR-α, and downregulated sterol regulatory element-binding protein-1 and fatty acid synthase, thereby enhancing lipid metabolism[147]. Additionally, Yan et al.[148]observed a rise in lactic acid and a decrease in citric acid during the fermentation process. Recently, new non-thermal technologies, such as selenium processing, have emerged. For example, our previous study found that selenium processing markedly increased total carbohydrate and soluble dietary fiber content while decreasing protein and insoluble dietary fiber content in Cordyceps militaris[149]. It also enhanced the biosynthesis of secondary metabolites, such as terpenoids and alkaloids. The application of these new technologies not only preserves food quality and enhances flavor but also allows for adjusting the nutritional composition of food to meet enhanced functional requirements.
-
Gut microbial-associated metabolites are systematically categorized into three groups: de novo synthesis, microbial dietary metabolism, and host metabolic contributions. The beneficial role and potential mechanism of novel gut microbial metabolites derived from natural products in chronic diseases are becoming clear. Furthermore, gut microbiota and their associated metabolites provide valuable insights into how food processing influences nutritional value and health benefits. However, the intricate diversity and complexity of these metabolites make the precise molecular mechanisms underlying their effects still poorly comprehended. To address this, future studies should aim to: (i) accurately elucidate the entire production process of metabolites; (ii) clarify the spatial and temporal efficacy targets of metabolites; and (iii) comprehensively understand the regulatory patterns of different types of metabolites in the body. Achieving these goals in future research will pave the way for deeper exploration of gut microbial metabolites and their health functions, providing more insightful comprehension and innovation in this field.
This work was supported by the Guangdong Basic and Applied Basic Research Foundation (2023A1515010744), National Natural Science Foundation of China (32202014), GDAS' Project of Science and Technology Development (2022GDASZH-2022010101), and State Key Laboratory of Applied Microbiology Southern China (SKLAM002-2022).
-
The authors confirm their contribution to the paper as follows: writing - original draft: Huang A; visualization: Huang A, Chen M; writing - review & editing: Ding Y, Zhu Z, Thanuphol P, da Cruz LL, Xie Z; supervision: Ding Y, Zhang F, Zhu Z; resources: Wu Q, Ding Y, Zhu Z; project administration: Ding Y, Zhu Z; funding acquisition: Wu Q, Ding Y, Zhu Z. All authors reviewed the results and approved the final version of the manuscript.
-
All data generated or analyzed during this study are included in this published article.
-
The authors declare that they have no conflict of interest.
- Copyright: © 2024 by the author(s). Published by Maximum Academic Press on behalf of China Agricultural University, Zhejiang University and Shenyang Agricultural University. This article is an open access article distributed under Creative Commons Attribution License (CC BY 4.0), visit https://creativecommons.org/licenses/by/4.0/.
-
About this article
Cite this article
Huang A, Wu Q, Thanuphol P, da Cruz LL, Xie Z, et al. 2024. Gut microbiota-associated metabolites in metabolic diseases and their impact from food processing. Food Innovation and Advances 3(4): 438−448 doi: 10.48130/fia-0024-0038
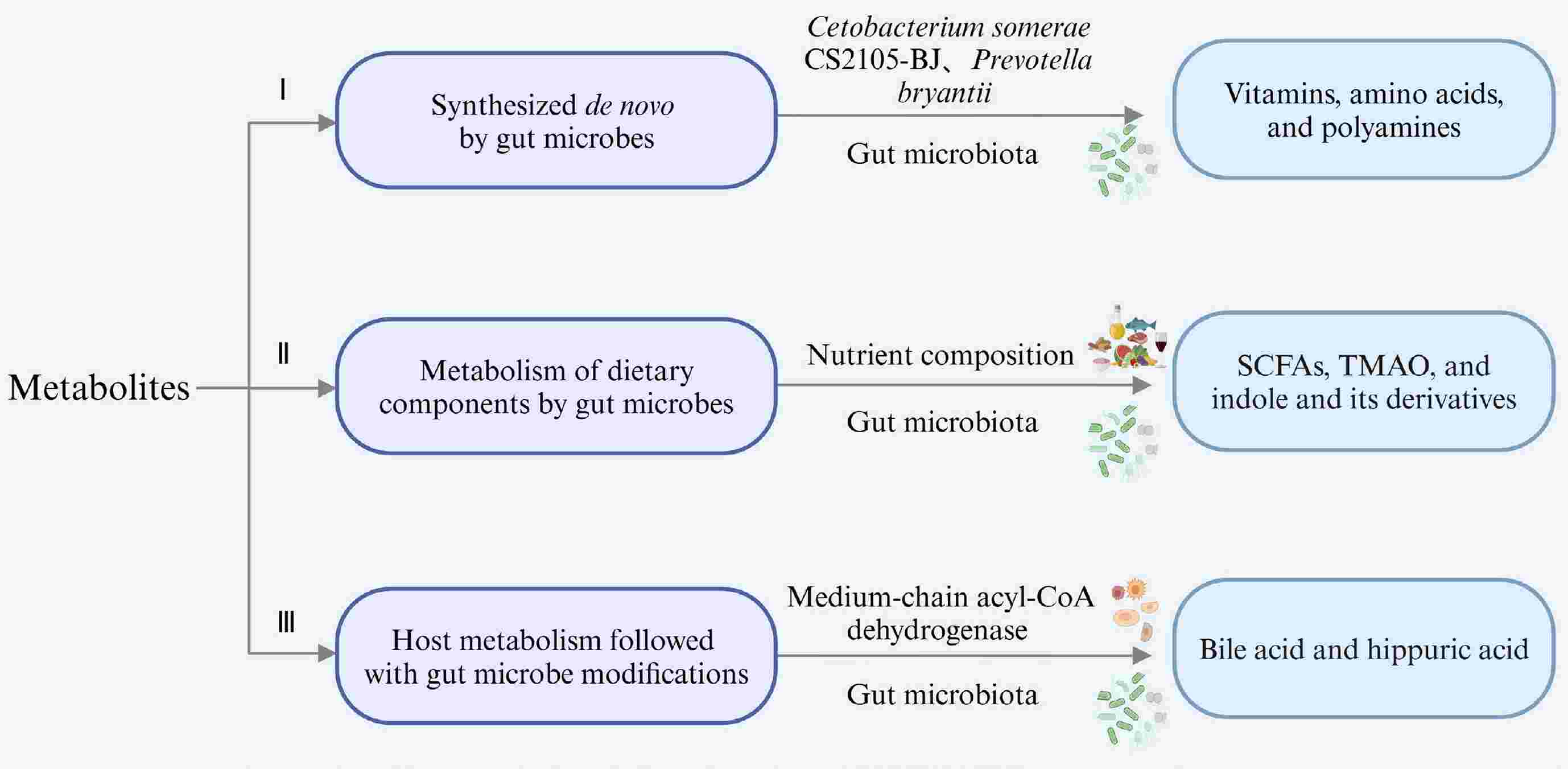