-
Bacteria are remarkably able to adapt their requirements for existence in various situations. One of the most significant microbial properties that promote these adaptations is the capacity of the microbe to create biofilms, as it enables adaptability to challenging environmental conditions. In recent decades, there has been a significant endeavor to enhance our comprehension of microbial biofilms. These biofilms are characterized as intricate and well-structured biological communities that are embedded within a highly hydrated extracellular polymeric matrix which contains various functional groups and organic biomolecules (Fig. 1). Proteins, carbohydrates, and nucleic acids in biofilm cells are known to be secreted into the matrix[1−3]. These biofilms could form on moist surfaces, whether biotic or abiotic. Biofilms are frequently observed in the food arena[4,5] and pose a significant problem due to their ability to attach to multiple surfaces, such as metal, plastic, glass, wood, soil particles, and food ingredients. The adherence of bacteria to food items or surfaces that come into touch with products results in financial losses and an increased likelihood of bacterial foodborne illnesses. Multiple lines of biochemical, structural, and proteomics investigations have also revealed that microbial biofilms are heterogeneous, and metabolically active[1,6−10]. Bacteria residing in biofilms provide survival benefits to their constituents by shielding them from environmental stressors such as UV radiation, dehydration, and exposure to antimicrobial and sanitizing agents[11]. Consequently, the eradication of these bacteria is a significant problem. In this mini-review, the negative impacts of biofilm in the food industry, factors that influence biofilm formation, and biofilm control strategies in the food industry are discussed.
-
The adherence of bacteria to surfaces in the food-contact surfaces, leading to the formation of biofilms, carry significant implications. The presence of organized microbial communities in food processing plants acts as a storage area for bacteria and can potentially contaminate raw materials and finished products at several phases of food production[12]. Furthermore, biofilms might result in food spoilage, financial losses, decreased product shelf life, or the spread of diseases. Biofilm could form on both food-contact surfaces and food products causing alteration of food quality (Fig. 2).
The initial publication on foodborne bacterial biofilm described the adhesive characteristics exhibited by Salmonella sp. Subsequently, numerous bacteria have been identified as capable of forming biofilms within the food industry environment. These bacteria include Yersinia enterocolitica, Listeria monocytogenes, Staphylococcus spp., Campylobacter jejuni, and Salmonella spp.
Salmonella enterica is a bacterium commonly associated with foodborne illness outbreaks in the food industry. It commonly spreads through the consumption of contaminated food, especially of animal origins like eggs, meat, and poultry. Infections caused by this pathogen can lead to gastroenteritis, by symptoms such as diarrhea, abdominal cramps, and fever. Fatima and her colleagues[13] documented the occurrence and antibiotic resistance profile of Salmonella found in uncooked beef originating from Lahore, Pakistan. Salmonella was found in 57 samples, with predominant strains Typhimurium, Typhi, and Enteritidis. The isolates showed significant resistance to various antibiotics, with erythromycin showing 100% resistance.
L. monocytogenes is frequently present in food processing environments and has previously been identified in both meat and dairy processing facilities. This microbe can quickly and securely attach to non-reactive surfaces and can remain in a stationary state for an extended duration. Nunes et al.[14] reported that 50% of Portuguese dairy farms tested positive for L. monocytogenes. Meanwhile, Beshiru & Uwhuba[15] found that all L. monocytogenes isolates in locally processed fermented foods in Ethiope West, Delta state, Nigeria were resistant to at least two types of antibiotics. Additionally, the multiple antibiotic resistance (MAR) score was equal to or greater than 0.22.
Staphylococcus aureus is a bacterium that is frequently linked to foodborne illness in the food industry. Food contamination can occur in multiple ways, including raw ingredients, food handlers, and inadequate hygiene practices. The production of toxins by this organism can result in food poisoning, primarily by the release of enterotoxins[16]. This can lead to symptoms such as nausea, vomiting, and diarrhea. In 2021, Seow et al.[17] found that S. aureus was present in 95% of food handlers and 50% of cooked food sold at Grade A, B, and C food premises in Klang Valley, Malaysia. A total of four methicillin-resistant S. aureus (MRSA) bacteria, accounting for 8.0% of the samples, were found in the cooked food samples. Furthermore, over 57% of the samples exhibited resistance to penicillin-G.
-
The adherence of pathogenic bacteria to surfaces used in meat processing, followed by the production of biofilms, presents a significant risk to meat products. This risk manifests in the form of reduced shelf-life and the potential for disease transmission during the processing and transportation stages. The main origins of germs that cause meat spoilage are animal intestines obtained after slaughter and cross-contamination that occurs during processing, transportation, and storage. Around 90% of microorganisms in the meat industry form biofilms, leading to an annual financial loss of US
150 million in the sector. L. monocytogenes and E. coli are regarded as the most perilous microorganisms for the meat industry due to their ability to endure and proliferate even in low temperatures[18]. Additional microbes that have been recognised as capable of generating biofilms in dairy products include Citrobacter, Bacillus, Aeromonas, Staphylococcus, Streptococcus, Pediococcus, Plesiomonas, Klebsiella, Proteus, Alcaligenes, Enterobacter, Moraxella, and Shigella species.$ The occurrence of biofilms in dairy processing plants presents a significant risk to the quality and shelf-life of dairy products. Several factors, such as prolonged processing time, intricate processing systems, delayed transportation, and distribution, may exacerbate the issue of biofilm formation[19]. This would result in the deterioration of milk product quality and an elevated risk of disease outbreaks. Biofilm-producing microbes possess the capacity to cause deterioration on a wide range of surfaces, including regeneration portions of pasteurizers, refrigerated tanks, milking systems, floors, conveyor chains, walls, steps, drains, and floors. These surfaces act as bases for the development of biofilms.
-
Various factors greatly influence the initial cell attachment in biofilm formation in the food processing sector (Fig. 3). Hydrophobicity, electrical charge, cation bridging, roughness, and topography are examples of surface characteristics[20,21]. There is no consensus on whether bacteria can adhere to surfaces that are either hydrophilic or hydrophobic. It is recognised that the various surface materials usually used in household kitchens or the food processing sector, such as granite, steel, glass, plastic, and marble, might contribute to the retention of foodborne pathogens[22]. In addition, the existence of food remnants or molecules, such as milk or beef proteins, on equipment used for food processing or handling can cause these substances to stick to the surface, resulting in the creation of a film that conditions the surface and potentially promotes the growth of biofilms[23]. Therefore, the presence of a food matrix can significantly impede the process of cleaning and disinfecting surfaces used in food preparation. Carrascosa et al.[24] also asserted that the constituents of the food matrix in food processing environments can impact the adherence of bacteria. Milk lactose enhances biofilm development in Bacillus subtilis by activating the LuxS-mediated quorum-sensing system, as demonstrated by Duanis-Assaf et al.[25]. Quorum sensing, the regulation of gene expression in response to cell-population density, is a key mechanism in both Gram-positive and Gram-negative bacteria. While Gram-negative bacteria primarily use N-acyl homoserine lactones (AHL) as signaling molecules, Gram-positive bacteria rely on peptides. These signaling molecules play a crucial role in regulating various physiological activities, including symbiosis, virulence, and biofilm formation[26].
-
To ensure food quality and safety, the adoption of Hazard Analysis and Critical Control Points (HACCP) and Good Manufacturing Practice (GMP) has been put in place. One of the initial measures to avoid and manage biofilms involve the identification of critical points where they have the potential to grow and expand. The susceptibility of microorganisms within biofilms to disinfectants and biocides can be up to 1,000 times lower than that of planktonic counterparts. This trait can be ascribed to multiple causes associated with the structure and functioning of biofilms, such as limited diffusion, anaerobic growth, physiological alterations caused by reduced growth rates, and the secretion of enzymes that break down antimicrobial compounds. The disinfectants commonly used by food industries, including phenolics, chlorine, iodine, hydrogen peroxide, and quaternary ammonium compounds, are not effective enough to eliminate biofilms[27].
Surface manipulation
-
A potential approach to managing biofilms involves the manipulation of surface chemical characteristics to inhibit cell adherence. Surfactants exhibit a broad range of structural properties, including both hydrophilic and hydrophobic structures. These properties enable surfactants to enhance their efficacy in physical cleaning processes by facilitating emulsification, penetration, spreading, foaming, and wetting[28]. Biosurfactants derived from a diverse range of bacteria, actinobacteria, and fungi have been extensively researched for their potential to inhibit biofilm formation. In a recent study, Kadiri et al.[29] showed that Bacillus aerius can produce a biosurfactant with an emulsification index (EI 24%) of 72.2% and a critical micellar concentration (CMC) of 60 mg/L. The biosurfactant molecules exhibited a strong attraction to specific proteins in Pseudomonas aeruginosa, including virulence factor LasA (3IT7), AHL Synthase LasI (1RO5), and transcription protein LasR (6MNV). The biosurfactant exhibited potent suppression against the biofilm of P. aeruginosa.
Edible coatings
-
Edible coatings are thin layers of edible substances applied to food surfaces to enhance preservation and safety (Fig. 4). They act as a barrier against oxygen, carbon dioxide, and moisture, which helps to slow down food spoilage and extend shelf life. They also help decrease lipid oxidation, which prevents rancidity and maintains food quality. According to Martins et al.[30], edible films and coatings are made from polymers and have a high potential to be combined with different additives in their structure and released after being stored. Active packaging enhances the longevity of food by either trapping (scavenging) or releasing (emitting) certain substances. Examples of commonly used edible coatings include alginate, pectin, arabic gum, dextran, carboxymethyl cellulose, and soy proteins[31,32]. Kaur et al.[33] stated that the inclusion of natural additives like glucosides, polysaccharides, phytosterols, phenolic acids, esters, carotenoids, tannins, alkaloids, anthocyanins, flavonoids, and terpenoids in edible films and coatings enhances their value by altering their physical, functional, and bioactive properties. Das et al.[34] examined the impacts of caraway oil and chitosan nanoemulsion coating (CH-CANE) on bananas. The researchers discovered that the CH-CANE nanoemulsion exhibited a nanometric size, low polydispersity, great stability, high whiteness, and a neutral pH. The CH-CANE nanoemulsion exhibited strong antibacterial and antibiofilm properties against E. coli and S. typhi. The application of the CH-CANE coating enhanced the quality and safety of bananas by decreasing deterioration and spoiling factors while simultaneously boosting antioxidant enzyme activity. Meanwhile, Johnson et al.[35] examined the impact of chitosan edible coating infused with medicinal leaf extracts on the antibiofilm and antibacterial properties against specific strains of Salmonella spp. and E. coli that were obtained from chicken samples. The combination of chitosan with M. piperita and P. amboinicus (ECMO) showed a biofilm inhibition percentage of up to 96% against both Salmonella spp. and E. coli. During the investigation of shelf-life, ECMO effectively reduced the proliferation of pathogens throughout a 15-d storage period. Table 1 summarizes previous works on edible coatings.
Table 1. Summary of previous works related to edible coatings containing antibiofilm agents.
Antibiofilm agents Edible coatings Foods Efficacy Authors Quercetin Pectin Apples The prepared edible coatings form a protective barrier over the surface of apples, effectively resisting bacterial infection and extending shelf life to 10 d while maintaining good commercial quality Du et al.[36] Pectin Corn starch Mozzarella cheese The pectin coating over mozzarella cheese increases its shelf life from 7 to 21 d. Tripathi & Mishra[37] ZnO nanoparticles Chitosan/gum
arabic (CH/GA)Banana CH/GA/ZnO coating maintains freshness of banana for more than 17 d in comparison with the less than 13 d for the control banana at 35 °C and 54% relative humidity. La et al.[38] Lytic bacteriophages Chitosan Tomato Approximately 3 log differences in microbial levels between the control and the treatment samples. Amarillas et al.[39] 3% thyme and oregano essential oil Soy protein Fresh beef The coatings with 3% thyme and oregano EOs exhibits 2.86 and 2.59, 1.97 and 1.90, and 1.87 and 1.83 log CFU/g reductions in S. aureus, L. monocytogenes, and E. coli populations, respectively, as compared with the control. Yemiş & Candoğan[40] Antibiofilm-coated food-contact surfaces
-
Antibiofilm-coated food-contact surfaces incorporate substances that inhibit the growth of pathogenic microorganisms, reducing the risk of food contamination (Fig. 5). These coatings release antimicrobial chemicals that either destroy or inhibit the attachment of microorganisms on surfaces, preventing their proliferation and biofilm formation. Compared to conventional cleaning methods, antibiofilm coatings offer enhanced disinfection, providing a safer environment for food preparation and handling. By eliminating microbial growth on surfaces, antibiofilm coatings effectively reduce the risk of cross-contamination between food items and food contact surfaces. Valliammai et al.[41] conducted a study where they developed a polymeric antibiofilm coating using citral (CIT) and thymol (THY) as active components. This coating was applied to a titanium surface using the spin coating method. The use of atomic force microscopy (AFM) allowed for the observation of a uniform coating, while a surface profilometer was used to assess the reduced surface roughness and thickness of the coating. The antibiofilm coating exhibited a controlled release of CIT and THY over a period of 60 d. The antibiofilm coating successfully prevented MRSA adherence under laboratory conditions, and the antibiofilm properties of the coating were not influenced by plasma conditioning. Potential applications of polymeric antibiofilm coatings in the food industry have also been reported by Acosta et al.[42], Esteves et al.[43], Sarvari et al.[44], Ogawa et al.[45], and Massoumi et al.[46]. Meanwhile, DeFlorio et al.[47] found that stainless-steel surfaces coated with superhydrophobic properties were resistant to fouling when in contact with contaminated romaine lettuce leaves. These surfaces retained their non-wetting properties even when subjected to abrasion with sand or exposure to high-concentration surfactant solutions. They suggested that integrating superhydrophobic stainless-steel surfaces into food processing equipment, namely for cleaning and packing leafy green vegetables, can reduce the spread of harmful microorganisms in food manufacturing facilities. Superhydrophobic coatings are known to inhibit biofilm formation by limiting the physical access of water to their surfaces. Table 2 summarizes previous works related to antimicrobial surface coatings.
Table 2. Summary of previous studies related to antibiofilm coated food-contact surfaces.
Antimicrobial agents Surface materials Efficacy Authors Microfibrillated cellulose Glass Inhibits the growth of both Gram-negative and Gram-positive bacteria (E. coli and S. epidermidis) due to the intrinsic porosity and hydrophilicity. Qi et al.[48] Gold nanoparticles Latex Reduces biofilm formation by E. coli, 369 to 9.87 ± 5.57% at a AuR NP concentration of 0.5 ng mL−1; and at a dose of 25 ng mL−1 no bacterial growth can be detected. Piktel et al.[49] Zinc oxide and silver oxide nanoparticles Polyester Polyester surfaces embedded with zinc oxide and silver oxide nanoparticles show sufficient, controlled levels of nanoparticles released to avoid bacterial adhesion. Fontecha-Umaña et al.[50] Copper nanoparticles Mussel-inspired dendritic polyglycerol
(MI-dPG)Cu NP-incorporated MI-dPG surface coating shows efficient long-term antibacterial properties against E. coli, S. aureus, and kanamycin-resistant E. coli through an 'attract–kill–release' strategy. This coating also inhibits biofilm formation and shows good compatibility to eukaryotic cells. Li et al.[51] Curcumin-liposome-type polydiacetylene/phosholipid nanovesicles Silanized glass Incubation of E. coli and Bacillus cereus with nanovesicle-coated glass results in a 2.5 log reduction in their counts. Dogra et al.[52] -
Studies have been carried out on several nanomaterials to explore their possible use in biosensors, with the goal of enhancing analytical features such as limit of detection (LOD), miniaturization, reusability, and sensitivity. The nanostructures that are now accessible include nano-rods, nanoparticles, thin films, nanotubes, and nanofibers[53]. The significance of prompt and discerning detection techniques cannot be emphasized enough in the realm of food safety, given that a substantial fraction of foodborne pathogens possesses the capability to produce exotoxins, even at minimal levels and densities. The fusion of biomolecules with nanostructures is the essential foundation for nano-biorecognition.
The schematic representation of nanotechnology applications in the food industry is depicted in Fig. 6. Absorbance measurements, facilitated by nanosensors, allow for quantitative assessment of biochemical reactions within food samples, aiding in determining the presence and concentration of pathogenic biofilms. Additionally, nanosensors can be designed for YES or NO detection, providing binary outcomes regarding the presence of extracellular matrix biofilms in foods. Nanosensors play a crucial role in the food industry due to their considerable capability to quantify and detect trace amounts of organic molecules, microorganisms, and other pollutants[54]. Moreover, these devices possess the capability to demonstrate rapid response, heightened sensitivity, and the ability to recover and integrate arrays on a significant magnitude[55]. Nanosensors, capable of converting biochemical information into optical signals, have recently been developed for very sensitive and precise optical detection of biofilms at the nanoscale level. Microbiological detection often makes use of nanoprobes and nanozymes due to their versatile optical properties, which may be adjusted and tailored for bacterium sensing and chemical recognition. Pu et al.[56] stated that nanosensors are designed to specifically transfer chemical information from biomolecules in a microscopic setting into detectable signals. Optical nanosensors usually comprise fluorescent, surface-enhanced Raman scattering (SERS), and colorimetric nanosensors. Graphene-based materials are known to improve the detection effectiveness and efficiency of nanosensors and other detectors[57,58]. Song et al.[59] developed a graphene-modified microelectrode array sensor to monitor biofilm growth in situ. The simulation of anti-biofilm drug screening clearly showed that this method performed far better than its endpoint alternatives. In 2018, Liu et al.[60] developed a hybrid nanosensor that combines fluorescent colloidal graphene with E. coli-activated RNA-cleaving DNAzyme. This nanosensor is capable of detecting E. coli in complicated biological samples. The release of DNAzyme is triggered by the presence of E. coli targets. The use of conductive polymer in the advancement of nanosensors has also garnered significant interest[61]. Conducting polymers capture and confine the biochemical sensing elements, allowing for detection in a nanosensor. Polyethylene dioxythiophene (PEDOT) is a highly studied conductive polymer due to its exceptional electrical stability and high conductivity. The measurement of acetic acid bacteria by PEDOT : PSS/Graphene/Nafion composite has previously been reported[62]. In a recent work, Udowo et al.[63] studied the interactions among three AHL molecules, graphene oxide (GO), and ZnO nanoparticles within a conjugated poly(3,4-ethylenedioxythiophene) (PEDOT) film. The results showed that PEDOT/GO/ZnO effectively detected AHLs to a significant extent. Other nanosensors for biofilm detection are summarized in Table 3.
Table 3. Summary of previous works on nanosensors for biofilm detection in foods.
Methods Materials Analyte Detection Authors Fluorescence Polystyrene nanoparticles pH in biofilms A temperature-dependent decrease in pH over a 4-h period caused by the acidifying glucose metabolism of E. coli Kromer et al.[64]. Fluorescence Graphene oxide nanoparticles DNA aptamers in biofilms The fluorescence intensity increases from 83.97 to 139.6 (a.u.) for the GO–APT1 mixed solution and from 85.07 to 172.6 (a.u.) for the GO–APT2 mixed solution. Wang et al.[65] Field-efect-transistor (FET) array Silicon nanowires Glucose metabolites in biofilms A high correlation between glucose signals by the redox-reactive SiNW FET device to the solution’s transparency measurements Yeor-Davidi et al.[66] Localized surface plasmon resonance (LSPR) Gold nanoparticles Biofilm growth kinetics After 2 h, the Au NM LSPR substrate is completely covered with either bacteria or conditioning biomolecules, preventing further shifts in the local dielectric constant, and thus the LSPR signal starts to exhibit blue shifts. Funari et al.[67] Fluorescence Gold nanoparticles Overall biofilm physicochemical properties The sensor is composed of AuNP-fluorescent protein conjugates that are disrupted in the presence of biofilms. This disruption turns on the fluorescence and results in different colored fluorescence patterns for biofilm identification. Li et al.[68] Foods are also analyzed using nanosensors to detect mycotoxins and pathogens[69]. The utilization of traditional techniques for detecting pathogenic microorganisms in food appears to lack adequate efficacy. Nevertheless, the advent of novel approaches such as nanosensors has effectively addressed this issue by enabling the swift identification of pathogenic strains and the detection of their released toxins throughout all phases of food production. Fluorescent nanosensors have significantly transformed food packaging by enabling the production of various colors[70]. Various instruments have been developed to detect numerous substances and bacterial toxins in food packaging through the utilization of antibodies and nanowires[71].
-
In the realm of food safety and processing, the formation of biofilms on food-contact surfaces poses multifaceted challenges with significant implications. These organized microbial communities act as reservoirs for bacteria, posing risks of contamination to raw materials and processed products throughout various stages of food production. Moreover, biofilms not only contribute to food spoilage and financial losses but also diminish product shelf life and heighten the potential for disease transmission. The initial adhesion of cells during biofilm formation is affected by a range of surface properties, including hydrophobicity, electrical charge, and roughness. These properties have distinct effects on various types of surface materials widely found in the home and industrial environments. To mitigate these risks, innovative strategies such as edible coatings, and active packaging have emerged as effective interventions. Edible coatings serve as barriers against oxygen, carbon dioxide, and moisture, thereby prolonging food preservation, and quality. Additionally, antibiofilm coatings on food-contact surfaces integrate antimicrobial agents, curbing the proliferation of pathogens and minimizing cross-contamination risks. Furthermore, advancements in nanotechnology offer promising avenues for enhancing biosensing capabilities in food safety, enabling rapid and sensitive detection of foodborne pathogens. The integration of nanostructures with biomolecules underscores a pivotal shift towards nano-biorecognition, laying the groundwork for precision-driven approaches to safeguarding food quality and public health.
-
The authors confirm contribution to the paper as follows: conceptualization: Yahya MFZR; original draft preparation: Yahya MFZR, Hidayah Mohamad Nor N; review and editing: Mahat MM, Siburian R, Yahya MFZR. All authors have read and agreed to the published version of the manuscript.
-
Data supporting this work is available within the article.
-
The authors would like to thank Universiti Teknologi MARA, Malaysia and Universitas Sumatera Utara, Indonesia.
-
The authors declare that they have no conflict of interest.
- Copyright: © 2024 by the author(s). Published by Maximum Academic Press on behalf of Nanjing Agricultural University. This article is an open access article distributed under Creative Commons Attribution License (CC BY 4.0), visit https://creativecommons.org/licenses/by/4.0/.
-
About this article
Cite this article
Yahya MFZR, Hidayah Mohamad Nor M, Mahat MM, Siburian R. 2024. Edible coating, food-contact surface coating, and nanosensor for biofilm mitigation plans in food industry. Food Materials Research 4: e025 doi: 10.48130/fmr-0024-0016
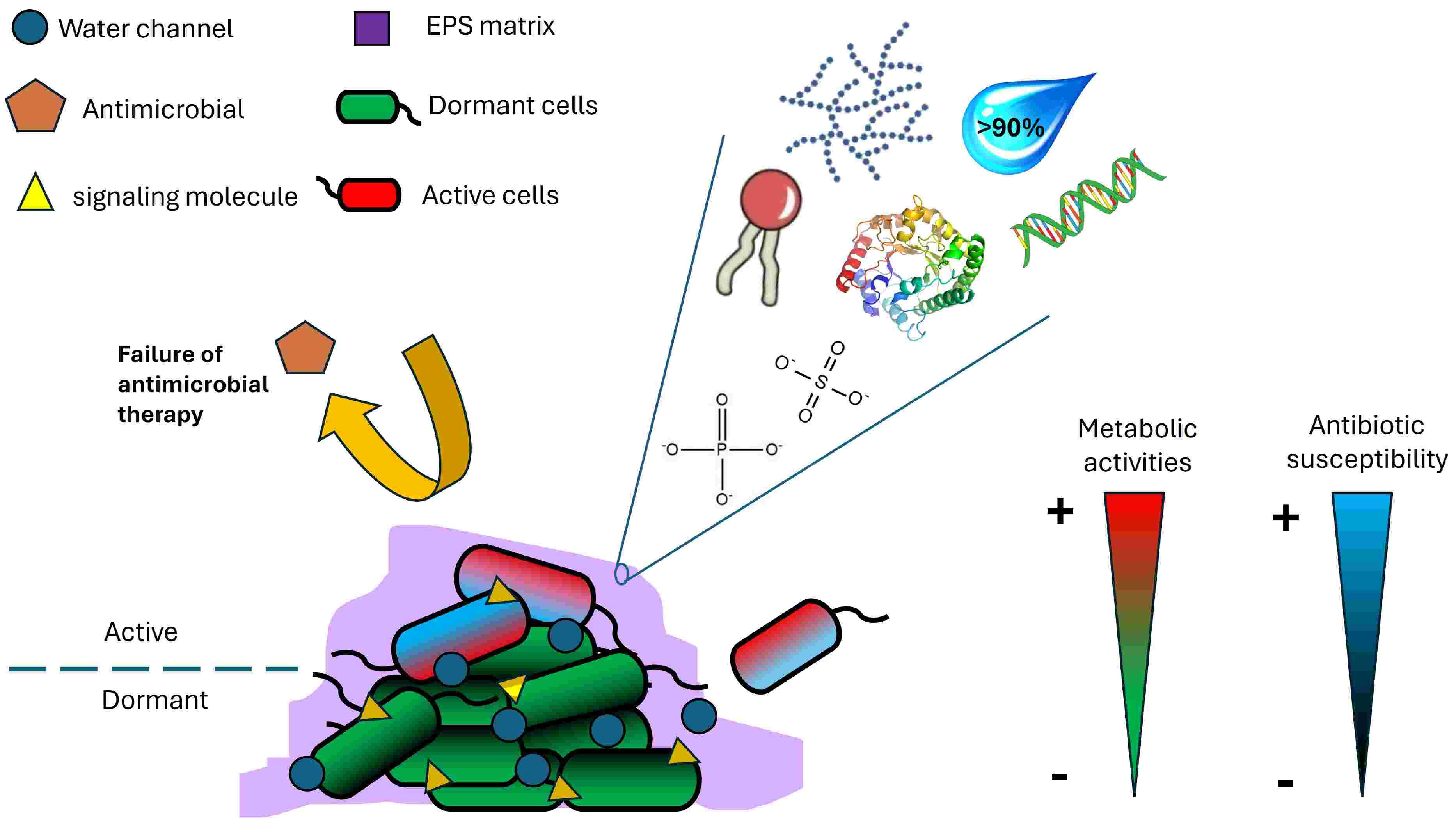