-
Gels are defined as viscoelastic formulations; they can be made by hydro (water) or oleogels (organic liquid). Hydrogels have several advantages, such as their ease of preparation, non-oily nature, and for topical applications good spreadability, increased hydration, cooling effect, and ease of removal. The main drawback is that they only load hydrophilic compounds. On the other hand, oleogels are easy to obtain and can load hydrophobic compounds. The main problem of the use of oleogels in the food and pharmaceutical industry is their oily nature[1−3].
The study of hydrogels and oleogels has been carried out for a long time, including several strategies to resolve problems, in this sense, the use of emulsion gel or emulgel has been introduced[4]. The emulgel usually is obtained by dispersing the liquid phase within the structured continuum phase[5]. The stability problem of the emulsion has been solved structuring disperse and continuum phase, this system has been named bigel[6]. Bigels have advantages of hydro and oleogels[7], also, the bigels, usually present better properties than either of the single gels[8] .
Different combinations of hydrocolloids, gelators and vegetable oil has been studied, more relevant examples of used hydrocolloids are the use of Guar gum[9,10], gelatin[11,12], sodium alginate[13,14], starch[15,16] and synthetic polymers (Poly(vinyl alcohol) (PVA) and Polyvinylpyrrolidone (PVP)[17].
Gelators for vegetable oil use sorbitan monopalmitate, policosanol, glyceryl stearate, beeswax, blend of monoglycerides[18,19], also, several vegetable oils have been used such as sunflower oil, olive oil and medium chain tryacylglycerides (MCT)[20, 21].
Usually, rheological and microscopic techniques are used to describe the mechanical properties of the obtained bigels, also, the modelling of behavior has been carried out usually by evaluate cohesiveness, firmness, adhesiveness, stickiness, viscosity, and creep recovery of bigels[3,10], however, less attention has been paid to evaluate the process parameters as mixing power, mixing time; with only the of ratio hydrogel/oleogel being well described[2,14] evaluate the influence of ratio hydrogel/organogel and mixing rate, Zhu et al.[22] demonstrate that the increase of oleogel fraction in bigels increase the firmness. An interesting approach to study several materials is the Cox-Merz rule. The so-called Cox-Merz 'rule' is the empirical relationship which has been found to be of great use in rheology. It was observed by Cox & Merz[23] that for many polymeric systems, correspondence occurred between the steady state shear viscosity, η, plotted against shear rate, and the magnitude of the complex viscosity, |η*|, plotted against angular frequency, ω, however, this rule has not been applied in polymer systems with cross-linked behavior or gelled systems, but several authors have demonstrated that their applicability in gel-like emulsions has been suggested in the entanglement systems they obey the Cox-Merz rule, also, if the mechanical spectra shows a weak-gel behavior, the network can survive small deformations, before breaking structure[24,25], reported that several emulsions under specific conditions fit well with the Cox-Merz rule.
To the best of our knowledge, no reports about the effect of mixing time and interaction of ratio hydrogel/organogel, mixing rate, mixing time and the applicability of Cox-Merz rule have been carried out for bigels.
-
Canola oil (61% monounsaturated, 32% polyunsaturated and 7% saturated) was purchased from the local supermarket (Durango, México) without further purification. Guar gum was purchased from Habacuq Comercializadora Química S.A de C.V. (Guadalajara, Jal, México). Myverol (18-04 PK) is a mixture of monoglycerides (mainly 49% glycerol monostearate, 48% glycerol monopalmitate and 3% calcium silicate) kindly provided by Kerry (SW food technology, SA de CV, Nuevo Leon, Mexico).
The surfactant PGPR (E 476), which is a complex mixture of partial esters of polyglycerol with linearly esterified polyricinoleic acid derived from castor oil, was purchased from Palsgaard (San Luis Potosí, Mexico). Polyoxyethylene sorbitan monostearate (Tween 60) was purchased from Sigma-Aldrich (Toluca, México).
Bigel preparation
-
Oleogels were prepared by dissolving myverol (10%, w/w) in canola oil (88.5% w/w) and PGPR as surfactant (1.5% w/w) under agitation at 80°C until complete solubilization following the method reported by Isaac Contreras-Ramírez et al[26]. The guar gum hydrogel (1%, w/w) was produced by dispersing the guar gum into distilled water under constant magnetic stirring at 80 °C for 1 h. Tween 60 was added to the water phase at 1.7% (w/w) under magnetic stirring. After that, the oil solution was cooled to 50 °C.
The bigels were prepared by incorporating the oleogel into the hydrogel and mixed at different ratios (67:33, 83:17; 85:15), followed by stirring at two different rates (600 and 800 rpm) for 20 min with an Ultra Turrax homogenizer (T25, IKA, Germany). Then the mixtures were cooled to room temperature, to trigger the structuring of oil phase and water phase. The obtained bigels were subsequently stored at 4 °C for further analysis.
Texture analysis
-
TA.TX plus texture analyzer (Texture Technologies Corp, New York, USA) was used to analyze the texture of the samples. Briefly, the instrument was calibrated (5 kg) and a penetration test was performed using a P20 cylindrical probe (aluminum probe, 20 mm diameter). A sample (50 g) of each formulation was placed in a container (35 mm diameter and 90 mm height) to a height of 35 mm. The probe penetrated at 5 mm·s−1 for 15 mm and returned to its initial position. From the force/time graph, the firmness value (maximum positive peak of the curve) was calculated following the method described by Pérez-Salas et al[27].
Thermal analysis
-
The differential scanning calorimeter DSC (TA Instruments, DE, USA) was used to analyze the thermal properties of bigel samples. About 10–15 mg of each bigel sample was sealed in aluminum pans with lids, an empty aluminum pan was used as a control. The thermal profile was determined in the cycle heating/cooling temperature into the range of 20 to 80 °C at a rate of 10 °C min−1 under nitrogen atmosphere, (flow rate of 10 mL·min−1).
Rheological analysis
-
Steady shear tests were carried out in a DHR-III rheometer (TA-Instrument, Delaware, USA), at a temperature of 25 °C, in a shear rate range of 0.1 to 100 s−1, with rough parallel plate geometry. The results were adjusted to the (Ostwald-de-Waale) power law model, using the ARES software (TA-Instrument, Delaware, USA). The evaluation of the linear viscoelasticity range was carried out in all the samples by means of a strain sweep in a range of 0.1% to 100%.
For oscillatory test It was carried out in a DHR-III rheometer (TA-Instrument, Delaware, USA), at room temperature and a geometry of rough parallel plate geometry 40 mm with 1,000 µm gap[28,29].
Creep–Compliance tests were performed on the bigels following the methodology proposed by Ojeda-Serna et al[30]. Ten Pa of stress was applied, and the response registered by 180 s, after that, force was retired, and compliance registered. The obtained results were modelling using the Burgers model.
Cox-Merz rule
-
The superimposition of the shear rate dependence of steady shear viscosity, and the frequency dependence of complex viscosity, η*(ω), at equal values of frequency and shear rate was carried out using the Cox–Merz rule[23]:
η∗(w)=η(γ)│w=γ (1) Where η* is complex viscosity, w is the frequency, η is the viscosity, γ is the shear rate.
This rule provides insight on the structure of materials. Deviation from Cox–Merz rule is an indication of structural heterogeneity in materials[31].
Statistical analysis
-
Data analysis was performed using the ANOVA test and mean comparison tests using the Tukey method (p < 0.05). The determination of parameters of the model used was obtained by non-linear estimation, using the Levenberg-Marquadt method, using the Statistica 12 software (StatSoft, Tulsa, OK, USA).
-
Results of the texture analysis are shown in Table 1. Firmness was defined as the maximum value of force in a plot of force vs time (positive peak), while the cohesiveness, was defined as the maximum value of the force in the negative peak arising during the movement of the probe upwards[27], this value could be used as the main parameter pointer of sample adhesion ability[5].
Table 1. Firmness and cohesiveness values of the bigels obtained at the different `proportions of hydrocolloid/oleogel at different agitation rates and mixing time.
Hydrocolloid/
oleogelAgitation
(rpm)Mixing
time (min)Firmness
(N)Cohesiveness
(N)67:33 11,200 5 1.19 ± 0.10ab −0.66 ± 0.001ab 67:33 11,200 7 1.48 ± 0.10ab −0.73 ± 0.030ab 67:33 15,600 5 1.60 ± 0.03b −0.76 ± 0.010b 67:33 15,600 7 1.55 ± 0.18ab −0.69 ± 0.120ab 83:17 11,200 5 0.88 ± 0.04ª −0.43 ± 0.020ab 83:17 11,200 7 0.92 ± 0.16ab −0.42 ± 0.090ª 83:17 15,600 5 1.02 ± 0.12ab −0.48 ± 0.010ab 83:17 15,600 7 1.03 ± 0.11ab −0.48 ± 0.040ab Data shown are the mean of three replicates ± standard deviation; different literals in the same column indicates statistical differences (p < 0.05, Tukey test). Thermal analysis
-
Results obtained by DSC analysis of bigels are showed in Table 2, the value of melting temperature was influenced only for the interaction of hydrogel/oleogel × mixing rate (p < 0.05), Highest melting point (Tm) (2.2 °C) was obtained at 67:33 hydrogel/oleogel ratio and 15,600 rpm.
Table 2. Calorimetric behavior (melting, crystallization) of the bigels obtained at the different proportions of hydrocolloid/oleogel at different agitation rates and mixing time.
Hydrocolloid/
oleogelAgitation
(rpm)Mixing time
(min)Melting point
(Tm) (°C)Melting enthalphy
(Kcal/mol)Crystallization point
(°C)Crystallization enthalphy
(Kcal/mol)67:33 11,200 5 0.9 ± 0.1 66.32 ± 17.43 −20.1 ± 0.3 75.99 ± 15.41 67:33 11,200 7 1.5 ± 0.2 133.15 ± 19.15 −16.6 ± 0.7 128.06 ± 11.74 67:33 15,600 5 1.9 ± 0.2 141.85 ± 13.35 −14.5 ± 0.2 129.40 ± 12.30 67:33 15,600 7 2.2 ± 0.6 164.05 ± 17.25 −13.8 ± 0.3 143.70 ± 13.80 83:17 11,200 5 1.5 ± 0.2 192.70 ± 23.80 −16.8 ± 0.1 163.40 ± 18.90 83:17 11,200 7 1.4 ± 0.1 201.10 ± 28.40 −17.0 ± 0.7 174.80 ± 17.40 83:17 15,600 5 0.9 ± 0.1 59.34 ± 15.75 −18.3 ± 0.2 86.50 ± 15.81 83:17 15,600 7 1.4 ± 0.3 195.6 ± 11.00 −15.7 ± 0.7 151.25 ± 20.15 Data shown are the mean of three replicates ± standard deviation; different literals in the same column indicates statistical differences (p < 0.05, Tukey test). Rheological behavior
Creep-Compliance
-
The Burgers model use Maxwell and Kelvin elements to study viscoelastic properties of the material, in short, G0 is a measurement of the sample resistance to deformation occurring immediately during the deformation profile. G0 is instantaneously recovered once the stress is removed. G1 is the contribution of the retarded elastic region to the total compliance; η0 is the residual viscosity or viscous flow of the system after suffering deformation, and η1 is the internal viscosity.[31]
J(t)=1Go+1G1[1−exp(−tG1η1)]+t/η0 (2) where G0 is the instantaneous elastic modulus of the Maxwell unit, G1 is the elastic modulus of Kelvin–Voigt. The dashpot of the Maxwell element represents the residual viscosity, η0, and the dashpot associated with Kelvin–Voigt is called the internal viscosity, η1. Parameters Go, G1. All parameters yielded values of r2 ≥ 0.94, which can be considered satisfactory (Table 3).
Table 3. Parameters of Burgers model of bigels at the different conditions of ratio hydrogel (H)/Oleogel (O), mixing rate, time of agitation.
Ratio H/O Rate (rpm) Time (min) Recovery (%) G0 Pa μ0 Pa s G1 Pa μ1 Pa s λ s R2 67:33 11,200 5 47.63 10.54 978.55 8.85 18.51 2.09 0.96 67:33 11,200 7 48.06 6.65 709.00 7.62 15.69 2.06 0.94 67:33 15,600 5 33.00 6.78 919.13 8.78 11.13 1.27 0.93 67:33 15,600 7 60.04 18.91 852.74 7.79 33.29 4.27 0.98 83:17 11,200 5 48.41 5.03 575.48 5.71 7.65 1.34 0.92 83:17 11,200 7 76.11 29.82 581.34 6.48 41.06 6.34 0.99 83:17 15,600 5 67.33 53.11 577.18 6.63 50.40 7.60 0.99 83:17 15,600 7 66.08 71.22 1402.51 20.12 174.16 8.65 0.99 Data shown are the mean of three replicates ± standard deviation. Cox-Mertz
-
Superposition of data from shear viscosity and complex viscosity was observed over the wide shear rate and/or frequency range for all samples, as illustrated in Fig. 1.
-
When the concentration of hydrogel increased from 63% to 87%, the firmness of bigel decreased (p < 0.05), major differences were observed between samples at low rate of agitation and high time of mixing, on the other hand, lower differences were observed for low rate of agitation and low time of mixing.
This behavior indicates that not only plasticing effect of surfactant molecules affect the firmness[27], but it is also important to evaluate process parameters involved in the obtention of bigels. Usually, surfactant increase Van der Waals interactions and hydrogen bonds between polymer strands, increasing the firmness of the gels. However, high rate of mixing and high time of mixing could be introduce air bubbles into the system, the presence of the new material into the system (air) affects the stability of the system, producing instabilities that diminish the firmness of the bigels[32].
Higher cohesiveness is an indication of the presence of tridimensional structure and then more consistency of the gel. Thus, in general, the higher presence of oleogel into the bigel (33% w/w) showed high cohesiveness. This could be attributed to the formation of an emulsion-like semisolid system with a more complex three-dimensional structure with droplets of oleogel dispersed in the hydrogel, more experiments are needed to probe the influence of the morphology in the texture behavior. Similar results have been reported by several authors[5,33], they observed higher hardness of emulsions and bigels when increasing the oil phase.
Thermal analysis
-
An increase in the melting temperature at higher amount of oleogel was reported by Singh et al.[34] for bigels made with sorbitan monostearate-sesame oil organogel and carbopol 934 hydrogel, however, they explained that higher proportion of oleogel increased the solid fraction and then higher temperatures were necessary to melt the bigel, however, they did not evaluate the influence of mixing rate, mixing rate apply a shear to the sample, results obtained indicate that high shear and high presence of oleogel produce more ordered systems than needing higher temperatures for melting.
Also, the melting enthalphy was influenced by the hydrogels/oleogel × mixing rate (p < 0.05), however, main effects (ratio hydrogel/oleogel, mixing rate) and the interaction of hydrogels/oleogel × mixing rate × mixing time showed statistical significance (p < 0.05), thus, the operational variables (mixing time, mixing rate) and the ratio of hydrogel/oleogel contribute to obtain specific melting temperature. The highest enthalpy of fusion was observed at the condition of lower amount of oleogel, lower stirring speed and longer stirring time. On the other hand, the lowest enthalpy of fusion was observed at the condition of higher amount of oleogel, lower stirring speed and lower mixing time. The higher value of melting entalphy could be related with the closer packing of the dispersed phase, related with the lower amount of oleogel, lower stirring rate and higher mixing time, that modify the size of the lipid particle[35], more experiments on particle size and morphology would help to clarifying this point.
In the present experiment, exothermic peak related with the crystallization was observed at the range of −13 to −20 °C (broader range than endothermic behavior), at the observed temperatures, the crystallization peak could be related with the canola oil in oleogel phase, this behavior is opposite of that reported by Singh et al.[10], who claim that the crystallization behavior of bigels is related with the presence of the gellators, however, in the present experiment, the crystallization of Myverol was around 52 °C, thus, the crystallization behavior was related with the presence of the canola oil into the bigels. The obtained results are in agreement with the reports by Contreras-Ramirez et al.[36], who reported for gelled emulsions made with canola oil, a crystallization temperature of −20 °C, like that found in the present work. There is a significant difference between melting and crystallization temperatures, however, the values of melting and crystallization temperatures do not show significant changes, this behavior has been reported by Habibi et al.[37].
Rheological behavior
-
All Parameters G0, G1, ƞ0, and ƞ1 showed a strong dependence of process parameters (hydrogel/oleogel ratio, mixing rate and mixing time; (p < 0.05), also, all statistical interactions were significative (p < 0.05). Higher G0 value was observed at lower amount of oleogel (17%) into the bigels and higher mixing rate. When more mixing time was used, independent of the proportion of hydrogel/oleogel, it obtained the highest value of G0, this behavior indicates lower deformation of the sample at the same imposed stress. Similar behavior was observed for G1. It is interesting that the contribution of G elements of Kelvin Voight and Maxwell, indicate that ratio G1/G0 was similar or lower than 1, indicating prevalence of the Maxwell element over the Kelvin Voight element, like the gelled emulsions reported by Ojeda-Serna et al.[30]. Major prevalence of the Maxwell model element was observed at lower amounts of oleogel, however statistical interaction (mixing rate and mixing time) is present indicating a complex system.
Higher values of recovery were observed with bigels with lower amount of oleogel (17%), also a strong statistical interaction was present (mixing time and mixing rate). Highest recovery (76%) was observed at lower proportions of oleogel, higher mixing time and lower mixing rate. On the other hand, lowest recovery (33%), was observed at high proportions of oleogel, high mixing rate and low time of mixing. High viscosity parameter was observed with bigels with high proportion of oleogel. The contribution of the viscous element to the compliance of bigels was higher than the contribution of dashpot elements, thus, the bigels obtained show a high viscosity with a lower structuration (dashpot), opposite to the behavior observed in organogels and gelled emulsions[26] (Table 3).
The empirical Cox–Merz superposition of steady-shear viscosity and oscillatory shear is consistent with topological entanglement interactions of individual species[38]. Also, the frequency sweep tests showed a significant frequency dependence of both moduli (Fig. 1) and the decrease in G0 with increasing amplitude of oscillation started at lower stresses, this behavior is usual in gels, especially when is they present weak topological interactions of polymer chains (founded into the hydrogel). In this sense, the gels are more sensitive to strain than solutions or dispersions.
The deviations from the Cox–Merz rule has been related to the specific polymeric and the entanglements founded in system with gel-like behavior[39]. Also, Vernon-Carter et al.[40] claim that the Cox-Merz rule usually fits well in a system showing a fluid-like or solid-like behavior.
In the field of bigels no reports about the use of Cox-Mertz rule have been carried out, however, Kwak et al.[41] use the Cox-Mertz rule to probe topical cream and ointments, finding that, these systems do not obey the rule. A system that fits the Cox-Merz rule indicated that the strain magnitude applied to the material does not provoke a structural decay.
Thus, the nature of the relationship displayed in Fig. 1 for the bigels were consistent with the Cox-Merz rule and indicates that these formulations are polymer dispersions. As expected, at high values of shear rate, the structure of the material is breaking, showing deviations from Cox-Merz rule at values higher than 30 s−1 of shear rate, however, at lower values of shear rate the Cox-Merz rule described very well the obtained bigels. Few examples of of emulsions that have been modelled by the Cox-Merz rule, have been found in the literature, Murillo-Martínez et al.[42] reported a multiple emulsion that fits very well to the Cox-Merz rule, and Medina-Torres et al.[43] indicate that highly structured systems did not obey this rule, opposite to the behavior of a polymeric dispersion, also, the use of master curves or time-temperature superposition approaches[44] could improve the knowledge about the bigels mechanical behavior.
-
Mixing time, mixing rate and rate of hydrogel/oleogel influence mechanical properties of bigels made with canola oil, myverol, guar gum and water. Better mechanical properties were obtained at lower mixing time, higher mixing rate and low proportion of oleogel in the bigels. The Cox-Merz rule described the complex viscosity and shear viscosity at low frequencies and shear rate in bigels.
-
JDMM acknowledges Conacyt for their scholarship.
-
The authors declare that they have no conflict of interest.
- Copyright: © 2023 by the author(s). Published by Maximum Academic Press on behalf of Nanjing Agricultural University. This article is an open access article distributed under Creative Commons Attribution License (CC BY 4.0), visit https://creativecommons.org/licenses/by/4.0/.
-
About this article
Cite this article
Mata-Mota JD, Gallegos-Infante JA, Pérez-Martínez JD, Rocha-Guzmán NE, González-Laredo RF. 2023. Effect of hydrogel/oleogel ratio, speed and time of mixing, on the mechanical properties of bigel materials and the application of Cox-Merz rule. Food Materials Research 3:24 doi: 10.48130/FMR-2023-0024
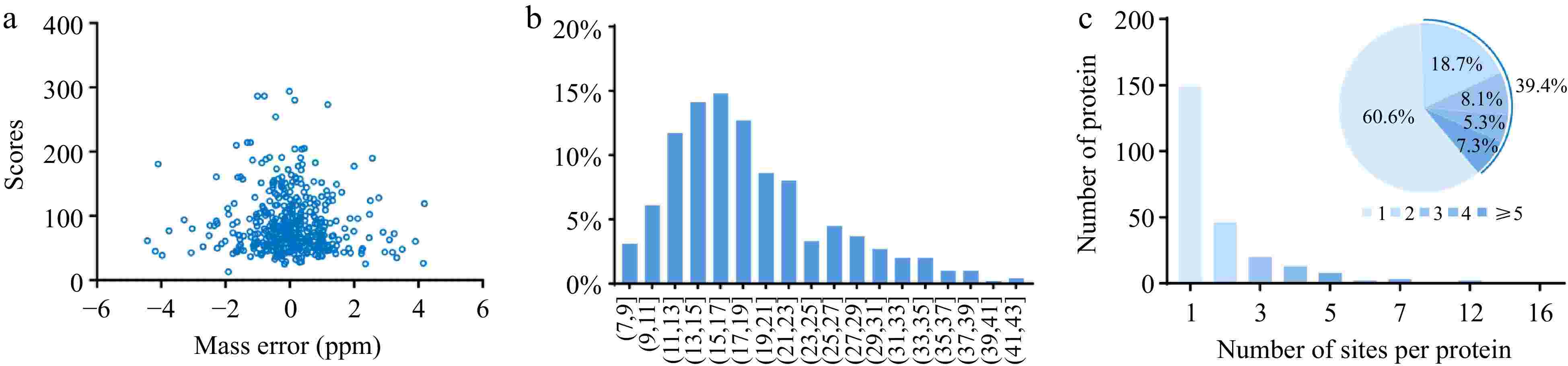