-
Hypertension is one of the major cardiovascular diseases and it has become a major menace to public health. The worldwide index has shown that more than one-quarter of the world population has hypertension[1]. Hypertension and its accompanying complications can cause morbidity and mortality[2]. Interestingly, hypertension is a multifactorial pathology that involves alteration in the activity of the renin-angiotensin-aldosterone system (RAAS)[3], cholinesterase[4], arginase[5], inflammation[6], chronic inflammation causes oxidative stress[7] and endothelial dysfunction[8]. Also, oxidative stress plays a significant role in the manifestation of hypertension by causing the endothelial system to malfunction, thereby impairing nitric oxide production and its vasodilation process[9]. In general practice, hypertension is managed through the use of antihypertensive medications which often cause side effects such as coughing, erection problems and sleep issues[10].
Nowadays, natural products have been used as a frontline alternative therapeutic agent for the management of hypertension. This may have been a result of their availability as well as little or no side effects following use. Remarkably, medicinal foods have become a popular alternative source of therapy for the management of various health-related issues, and have a proven scientific basis to support their folklore claims. However, vegetables which form a greater proportion of the human daily diet, have been referred to as protective foods because they are endowed with diverse bioactive compounds which offer varying health benefits such as modulation of immunity, prevention of gastrointestinal disorders, cardiovascular diseases, cancer, diabetes, and other chronic diseases. In Africa, a lot of vegetables are used as part of daily meals, not because of their nutritional properties or edibility only but because most of these vegetables provide health-enhanced benefits[11].
African wild lettuce (Launaea taraxacifolia) is an annual West Tropical Africa herb commonly known as wild lettuce. Wild lettuce is among some of the vegetables that are on the verge of extinction. Wild lettuce is wide spread in some African countries such as Nigeria, Ghana and Sierra Leone. Wild lettuce leaves are commonly consumed as soup, salad or eaten as spinach[12]. A number of research studies have indicated that wild lettuce leafs have antioxidant properties[13], protective effects against DNA and kidney damage[14] antimicrobial and antiarthritic effects[15] and hypolipidaemic properties[14, 15]. Also, a study has shown that wild lettuce leaf has been used for the management of liver diseases, dyslipidemia, and diabetes[13, 14]. In the same vein, wild lettuce leaf has a diverse amount of macro and micronutrients which make it a good choice of vegetable for healthy living.
African eggplant (Solanum macrocarpon), also known as efo igbagba in southwestern Nigeria is a local leafy vegetable that is cultivated mainly for its leaves. Unlike the other solanum varieties, its bitter taste has made the fruits less edible[16]. Macrocarpon has many benefits. It is readily available, cheap, and nutritious and it can be used to prepare soup. Kaushik et al.[17] maintained that Solanum macrocarpon could be used to treat tuberculosis, convulsion and boost infertility and insomnia in women in the traditional way. The leaves can be boiled to extract the juice which can be used to alleviate jaundice, asthma and whooping cough[18]. Also, infusion from Solanum macrocarpon leaves have been used for the treatment of some ailments such as of some diseases such as liver disease, dyslipidemia, and diabetes[19,20]. However, as reported in folk medicine, these vegetables exhibit cardioprotective properties with a dearth of information on their underlying mechanisms through which acclaimed health benefits could be achieved. Therefore, the present study sought to unravel the underlying mechanism through which wild lettuce and African eggplant leaves exhibit antihypertensive properties in L-NAME-induced hypertensive rats.
-
The African wild lettuce leaves (Launaea taraxacifolia) and African eggplant leaves (Solanum macrocarpa L.) were obtained from a farm settlement in Akure, Ondo state, Nigeria. The vegetables were authenticated by Mr Momoh, a Forest Biologist at the herbarium, Federal University of Technology, Akure (FUTA), Nigeria. Then, the leafy part was removed, rinsed, air-dried, pulverized and sieved into powdery form. The aqueous extract was prepared by dispersing 500 mg of each sample in 250 mL of distilled water and vigorously shaking for 24 h using a HY-4 speed governing multipurpose oscillator (HINOTEK, Ningbo, China). The mixture was initially filtered using a sieving mesh while subsequent filtration was carried out with Whatman filter paper and the obtained filtrate was lyophilized to obtain a powdered form of the samples.
Chemicals and reagents
-
Chemicals such as N(ω)-nitro-L-arginine methyl ester (L-NAME), Hippuryl-L-Histidyl-L-Leucine (HHL), thiobarbituric acid (TBA) and other chemicals and reagents used for this study were of analytical grade, while all-glass distillers used for water distillation were of high grade
Experimental grouping and protocol
-
Healthy male Wistar strain rats (number = 10, weight: 180–200 g, age 11–12 weeks) were sourced from the University of Ibadan (Nigeria). Thereafter, the institution's research committee granted ethical clearance for the utilization of experimental rats. The National and Institutional guidelines for animal protection and welfare were followed strictly. The rats were maintained at room temperature (25 °C) with free access to food and water. They were allowed to adapt to their new environment for 2 weeks prior to the commencement of induction and treatment. Thereafter, acclimatized rats were administered with 40 mg/kg body weight (BW) except for group 1 rats and the treatment regime lasted for 14 d. Experimental rats were fed with animal feed with six rats per group.
Experimental design
-
Group I: Normotensive;
Group II: Hypertensive (HYP) rats;
Group III: HYP rats administered with captopril [10 mg/kg body weight (BW)];
Group IV: HYP rats administered with 250 mg/kg BW of wild lettuce;
Group V: HYP rats administered with 500 mg/kg BW of wild lettuce;
Group VI: HYP rats administered with 250 mg/kg BW of African eggplant;
Group VII: HYP rats administered with 500 mg/kg BW of African eggplant daily
Thereafter, the experimental rats were sacrificed, tissues of interest were collected, homogenized and the homogenate was used for biochemical evaluations.
-
The heart and kidney ACE activity was determined as described by Cushman & Cheung[21]. The substrate [hippuryl-histidylleucine (Bz-Hip-HisLeu)] was purchased from Sigma Aldrich. The amount of cleaved hippuric acid from hippuric-histidyl-leucine was measured by the enzymatic method. Sample (50 μL) and 150 μL of 8.33 mM of hippuric-histidyl-leucine (Bz-Hip-His-Leu) in 125 mM Tris-HCl buffer (pH 8.3) were incubated at 37 °C for 30 min. After incubation, the reaction was arrested by adding 250 μL of 1 M HCl. The Gly–His bond was then cleaved, and the hippuric acid produced by the reaction was extracted with 1.5 mL ethyl acetate. Next, the mixture was centrifuged to separate the ethyl acetate layer; then, 1 mL of the ethyl acetate layer was transferred to a clean test tube and evaporated. The residue was redissolved in distilled water, and its absorbance was measured at 228 nm. The plasma ACE activity was expressed as μmol HHL cleaved/min
Determination of arginase activity
-
Arginase activity in the heart and kidney tissue was determined by measuring the rate of urea production using α-isonitrosopropriophenone (9% in absolute ethanol) as previously described by Kaysen & Strecker[22]. Briefly, 50 μl of samples were added into 75 μl of Tris-HCl (50 mmol/l, pH 7.5) containing 10 mmol/l MnCl2 and was pre-incubated at 37 °C for 10 min to activate the enzyme. The hydrolysis reaction of L-arginine by arginase was performed by incubating the mixture containing activated arginase with 50 μl of L-arginine (0.5 mol/l, pH 9.7) at 37 °C for 1 h and was stopped by adding 400 μl of the acid solution mixture [H2SO4 : H3PO4 : H2O = 1:3:7 (v/v/v)]. For calorimetric determination of urea, α-isonitrosopropiophenone (25 μl, 9% in absolute ethanol) was then added and the mixture was heated at 100 °C for 45 min. After placing the sample in the dark for 10 min at room temperature, the urea concentration was determined spectrophotometrically by the absorbance at 550 nm. The amount of urea produced was used as an index for arginase activity. The arginase activity was expressed as μmol urea produced/min/mg protein.
Determination of acetylcholinesterase activity
-
The AChE enzymatic assay was determined according to the method of Ellman et al.[23]. The reaction mixture (2 ml final volume) contained 100 mM K+-phosphate buffer, pH 7.5 and 1 mM 5,5′-dithiobisnitrobenzoic acid (DTNB). The method is based on the formation of the yellow anion, 5,5′-dithio-bis-acid-nitrobenzoic, measured by absorbance at 412 nm during 2 min incubation at 25 °C. The enzyme (40–50 mg of protein) was pre- incubated for 2 min. The reaction was initiated by adding 0.8 mM acetylthiocholine iodide (AcSCh) for acetylcholineterase assay. All samples were in triplicate readings and the enzyme activities were expressed in units/mg of protein.
Nitric oxide level (NOx) determination
-
NOx content was estimated in a medium containing 70 μl of the sample, 70 μl of 2% vanadium chloride (VCl3) in 5% HCl, 70 μl of 0.1% N-(l-naphthyl) ethylenediamine dihydrochloride and 2% sulphanilamide (in 5% HCl) in 1:1 ratio. After incubating at 37 °C for 60 min, nitrite levels, which correspond to an estimative level of NOx, were determined spectrophotometrically at 540 nm, based on the reduction of nitrate to nitrite by VCl3[24]. The nitrite and nitrate levels were expressed as nanomoles of NOx/mg protein.
Estimation of the total thiol level
-
The total thiol level was determined in the tissue (heart, kidney and lung) and homogenates according to the method previously described by Ellman[25]. The reaction system was made up of 170 mL of 0.1 M potassium phosphate buffer (pH 7.4), 20 mL of sample, and 10 mL of 10 mM DTNB. At the end of 30 min incubation at room temperature, the absorbance was measured at 412 nm. A standard curve was plotted for each measurement using reduced glutathione (GSH) as a standard and the results were expressed as mmol/mg protein.
Determination of tissue lipid peroxidation
-
The lipid peroxidation assay was carried out using the modified method of Ohkawa et al.[26]. Briefly, 300 μl of tissue (plasma, heart, kidney and lungs) homogenate, 300 μl of 8.1% SDS (Sodium dodecyl sulphate), 500 μl of Acetic acid/HCl (PH = 3.4) and TBA (Thiobarbituric acid) were added, and the mixture was incubated at 100 °C for 1 h. Thereafter, the thiobarbituric acid reactive species (TBARS) produced was measured at 532 nm and calculated as Malondialdehyde (MDA) equivalent.
Total protein determination
-
Protein was measured by the Coomassie blue method according to Bradford[27] using serum albumin as standard.
Data analysis
-
The values were expressed as mean ± standard deviation (SD). The mean differences in each group were analyzed by one-way ANOVA using Graph pad prism 5.0, followed by a posthoc test using Turkey s multiple range tests at the level p < 0.05.
Results
-
Figure 1 represents the heart and kidney ACE activity of normotensive rats, untreated hypertensive, and treated hypertensive rats. The result as presented showed that hypertensive rats had higher activity of ACE when compared with the normotensive rats. However, administration of captopril (20 mg/kg BW), wild lettuce (WL) and African eggplant leaf (AP) (250 and 500 mg/kg BW) respectively caused a reduction in the ACE activity when compared with the untreated hypertensive rats. Comparatively, WL administration had less activity ACE in hypertensive rats than AP.
Figure 1.
Effect of wild lettuce and African eggplant leaves extract on (a) lungs and (b) kidney ACE activity in L-NAME induced hypertension in rats. Values represent mean ± standard deviation (n = 6). * Significantly different when compared normotensive with hypertensive (p < 0.05). ** Significantly different when compared wild lettuce and African eggplant leaves extract-treated hypertensive with hypertensive (p < 0.05).
Also, Fig. 2 depicts the heart and kidney arginase activity of arginase of normotensive, hypertensive and treated hypertensive rats. Obtained results revealed an elevated arginase activity in the hypertensive rats when compared with normotensive rats. Thereafter, administration of captopril (20 mg/kg BW), WL and AP leaf extract (250 and 500 mg/kg BW) brings about a reduction in arginase activity in the treated hypertensive rats in comparison with the hypertensive rats.
Figure 2.
Effect of wild lettuce and Africa eggplant leaves extract on the (a) heart and (b) kidney arginase activity in L-NAME induced hypertension in rats. Values represent mean ± standard deviation (n = 6). * Significantly different when compared normotensive with hypertensive (p < 0.05). ** Significantly different when compared wild lettuce and African eggplant leaves extract-treated hypertensive with hypertensive (p < 0.05).
Furthermore, Fig. 3 depicts the activity of AChE in the heart and kidney of normotensive and untreated and treated hypertensive rats. As obtainable, untreated hypertensive rats had significantly (p < 0.05) higher AChE activity when compared with the normotensive rats. Meanwhile, administration of captopril, WL and AP leaf extract (250 and 500 mg/kg body weight respectively) caused the elevated AChE activity to be minimal in the treated hypertensive rats when correlated with untreated hypertensive rats.
Figure 3.
Effect of wild lettuce and Africa eggplant leaves extract on the (a) heart and (b) kidney acetylcholinesterase (AChE) activity in L-NAME induced hypertension in rats. Values represent mean ± standard deviation (n = 6). * Significantly different when compared normotensive with hypertensive (p < 0.05). ** Significantly different when compared wild lettuce and African eggplant leaves extract-treated hypertensive with hypertensive (p < 0.05).
Likewise, Fig. 4 depicts the level of nitric oxide (NO) in normotensive rats and hypertensive (untreated and treated). Interestingly obtained results showed that hypertensive rats had a declining level of NO in the plasma, heart, kidney and lungs when compared with the NO level of normotensive rats. Meanwhile, the plasma, heart, kidney and lungs NO level of treated hypertensive rats was observed higher with respect to what is obtainable for the untreated hypertensive rats. Nevertheless, WL-treated groups had higher NO levels than AP-treated groups.
Figure 4.
Effect of wild lettuce and Africa eggplant leaves extract on (a) plasma, (b) heart, (c) kidney and (d) lungs Nitric oxide level in L-NAME induced hypertension in rats. Values represent mean ± standard deviation (n = 6). * Significantly different when compared normotensive with hypertensive (p < 0.05). ** Significantly different when compared wild lettuce and African eggplant leaves extract treated hypertensive with hypertensive (p < 0.05).
Furthermore, heart, kidney and lung total thiol levels as presented in Fig. 5. As presented, untreated hypertensive rats exhibited reduced levels of total thiol in comparison with normotensive rats. Remarkably, hypertensive rats treated with captopril, WL and AP aqueous extract (250 and 500 mg/kg BW respectively) exhibited higher total thiol levels when compared to the untreated hypertensive rats. Comparatively, WL and AP at 500 mg/kg body weight enhanced total thiol levels significantly higher than 250 mg/kg body weight.
Figure 5.
Effect of wild lettuce and Africa eggplant leaves extract on the (a) heart, (b) kidney and (c) lungs total thiol level in L-NAME induced hypertension in rats. Values represent mean ± standard deviation (n = 6). * Significantly different when compared normotensive with hypertensive (p < 0.05). ** Significantly different when compared wild lettuce and African eggplant leaves extract-treated hypertensive with hypertensive (p < 0.05). β Not significantly different when compared wild lettuce and African eggplant leaves extract-treated hypertensive with hypertensive.
Also, Fig. 6 represents TBARS levels in the plasma, heart, kidney and lungs of experimental rats. As presented, normotensive and treated hypertensive rats had lower TBARS levels in plasma, heart, kidney and lung in comparison with untreated hypertensive rats.
Figure 6.
Effect of wild lettuce and African eggplant leaves extract on malondialdehyde level in (a) plasma, (b) heart, (c) kidney and (d) lungs of L-NAME induced hypertensive rats. Values represent mean ± standard deviation (n = 6). * Significantly different when compared normotensive with hypertensive (p < 0.05). ** Significantly different when compared wild lettuce and African eggplant leaves extract-treated hypertensive with hypertensive (p < 0.05). β Not significantly different when compared wild lettuce and African eggplant leaves extract-treated hypertensive with hypertensive.
Finally, Fig. 7 and Table 1 show the peaks on the chromatogram and corresponding bioactive compound constituents of wild lettuce (WL) and African eggplant (AP) leaves obtained using GC-MS analysis. As shown on the chromatogram, 12 bioactive compounds listed as follows: P-coumaric acid, cinnamic acid, Quercetin, Syringic acid, Kaempferol and others were quantified from wild lettuce and African eggplant leaves respectively as shown in Table 1.
Figure 7.
(a) GC-MS fingerprint of wild lettuce (Launaea Taraxacifolia) leaves bioactive constituents. (b) GC-MS fingerprint of African eggplant (Solanum macrocarpon) leaves bioactive constituents.
Table 1. Bioactive compound constituent of wild lettuce and African eggplant leaves.
S/N Compounds detected Molecular formula Phenolic content (mg/g) Wild
lettuceAfrican eggplant 1 1,2,3-Benzenetriol C6H6O3 0.04 0.02 2 Gentisic acid C5H11NO2 0.03 0.02 3 Benzoic acid, 4-hydroxy- C7H6O3 0.36 0.38 4 Cinnamic acid C12H16O2Si 1.22 1.31 5 Syringic acid C15H26O5Si2 1.07 1.03 6 Protocathecolic acid C16H30O4Si3 0.32 0.49 7 Kaempferol C27H44O6Si4 0.91 0.62 8 Quercetin C15H10O7 1.18 1.14 9 3-Caffeoyl quinic acid C34H66O9Si6 0.41 0.54 10 Cathecol C12H22O2Si2 0.44 0.54 11 Tyrosol C14H26O2Si2 0.01 0.02 12 P-coumaric acid C9H8O3 1.31 1.41 -
Vegetables constitute a critical portion of the human diet. WL and EP have been used by Nigerian local dwellers for the management of some diseases such as hypertension without scientific basis. Experimentally, administration of L-NAME at 40 mg/kg BW causes a hypertensive effect in normotensive rats[28,29] as well as a manifestation of oxidative stress[30]. Hypertension is a multifactorial pathology which involves alteration in the activity of some enzymes like ACE, arginase, cholinergic and purinergic enzymes as well as oxidative stress[31]. It is worth noting that one of the mechanisms through which L-NAME causes hypertension is via ACE up-regulation[32]. As presented (Fig. 1), the elevated ACE activity was drastically reduced after the administration of WL and AP aqueous extract to the L-NAME-induced hypertensive rats. It should be on a note that ACE causes hypertension via its catalytic effect on angiotensin I which result in the formation of angiotensin II, a potent vasoconstrictor[32]. In addition to the production of angiotensin II, ACE also enhanced the degradation of the potent vasodilator, bradykinin which eventually contribute to the hypertensive effect of ACE activity[33]. The WL and EP leaf extracts administrated caused a sharp decline in the activity of ACE in the hypertensive rats serving as an indicator for the anti-hypertensive protective mechanism of the selected vegetable. Likewise, the availability of bradykinin will be enhanced by reduced ACE activity since this will ensure the vascular-promoting effect of bradykinin[34]. This observed inhibition or reduction in the activity of ACE could be linked to the effect of diverse bioactive compounds in the WL and EP leaves. Studies have shown that hydroxyl, carboxylic, sulfurhydric or o-methylation groups of the plant bioactive compounds interact with Zinc II ion residues at the active site of the ACE protein which might cause an alteration in the active site resulting in the observed reduction in activity[35]. Research findings have shown that the dietary approach offers a protective effect against hypertensive effects[36,37]. Also, findings from this study agree with previous reports on the effect of bioactive compounds from vegetables on ACE activity[38,39].
Arginase play a prominent function in the development of hypertension via its negative direct effect on NO-level production. L-NAME administration significantly impairs NO production[40] via its inhibitory effect on endothelial nitric oxide synthase (eNOS). eNOS and arginase compete for available L-arginine for their respective bioactivity. In a hypertensive state, elevated arginase activity has been observed which implies that the majority of the available L-arginine will be utilized by arginase and this implies that the NO production by e-NOS will be impaired[41]. It should be noted that elevated arginase activity in hypertensive individuals and in rats’ models has been reported as high[34]. As presented, WL and EP leaves extract lower arginase activity in L-NAME-induced hypertensive rats, implying that L-arginine will be preserved for e-NOS for NO production.
The observed high NO level is possible due to a reduction in the enzymatic activity of arginase, thereby ensuring more NO availability for the vasodilatory processes. However, administration of aqueous extract reversed the L-NAME inhibitory effect on e-NOS, reduced the activity of arginase and ensure an ample amount of NO for vasodilatory processes. The observed effect on arginase activity and NO level further explained another antihypertensive mechanism of wild lettuce and African eggplant leaves[42,43]. Also, research findings have shown that AChE contributes to the onset of cardiovascular diseases such as hypertension. Elevated AChE activity causes a significant reduction in acetylcholine bioavailability causing constriction of smooth muscle[31]. The eNOS mediated smooth muscle vasodilation and relaxation role in conjunction with released acetylcholine (ACh) from the cholinergic nerves as reported by Kellogg Jr et al.[44]. ACh availability is subjected to the hydrolytic effect of AChE which hydrolyzes ACh to Acetate and choline[45]. The WL and EP leaves ability to reduce the activity of AChE in hypertensive rats could be a result of their phytochemical constituents as presented in Fig. 7 and Table 1.
Oxidative stress has been implicated in the development of hypertension justified by the presence of a well-established mechanism of cellular injury known as lipid peroxidation[46]. Also, administration of an aqueous extract of WL and EP leaves significantly enhanced the endogenous antioxidant status of the hypertensive rats as grossly considered by an enhanced level of total thiol level with a corresponding decrease in TBARS level in the treated hypertensive rats. The result of our study showed that L-LAME administration has the ability to promote oxidative imbalance adjudged by lipid peroxidation and drastically reduce the level of total thiol in the untreated experimental rats, the antioxidant defence mechanism potential of WL and EP leaves against oxidative stress was reflected in their ability to minimise TBARS level and elevate the level of total thiol in treated rats. This implies that the bioactive constituent of WL and EP leaves could prevent the rapid depletion of the antioxidant capacity of hypertensive rats. Vegetables have been reported to exhibit diverse antioxidant properties due to the presence of polyphenolic compounds[46,47].
In conclusion, this study has revealed some underlying mechanisms through which WL and EP leaves exhibits anti-hypertensive effect. Wild lettuce and African eggplant leaves reduced ACE, arginase and AChE activity and enhanced NO levels in hypertensive rats. Also, the occurrence of bioactive compounds such as P-coumaric acid, cinnamic acid, quercetin, syringic acid, Kaempferol and others may have responsible for the anti-hypertensive property and antioxidant effect. However, WL showed more antihypertensive potential compared to EP leaves, nonetheless, both vegetables have the ability to ameliorate hypertensive effects. Therefore, the vegetables selected for this study should be prevented from going into extinction.
-
The institution and Department of Animal Ethics Committee approved the use of experimental animals prior to the commencement of the study.
-
The authors declare that they have no conflict of interest.
- Copyright: © 2023 by the author(s). Published by Maximum Academic Press on behalf of Nanjing Agricultural University. This article is an open access article distributed under Creative Commons Attribution License (CC BY 4.0), visit https://creativecommons.org/licenses/by/4.0/.
-
About this article
Cite this article
Agunloye OM, Olawuyi EA, Oboh G. 2023. Modulatory effect of wild lettuce and African eggplant leaf extract on key enzymatic activity linked to hypertension in L-NAME induced hypertensive rats. Food Materials Research 3:7 doi: 10.48130/FMR-2023-0007
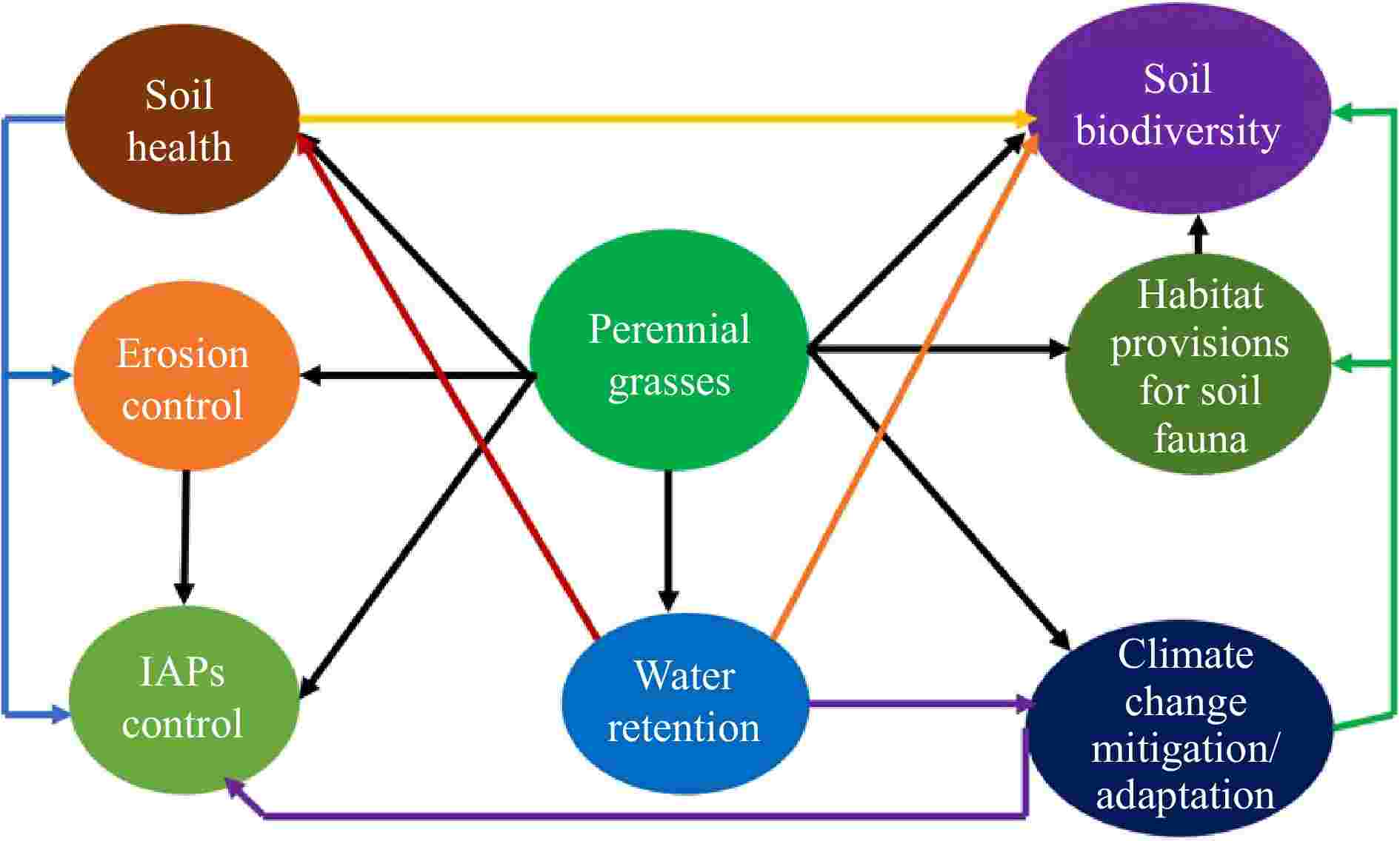