-
Akkermansia muciniphila has recently been recognized as a key commensal bacterium in the human gut, playing a pivotal role in modulating host metabolic functions and immune responses[1−3]. The correlation between its optimal abundance and the prevention of various metabolic diseases and immune dysfunctions show the necessity of maintaining balanced levels of this bacterial species for overall health. Mucins are a group of glycoproteins abundant in the gut mucosal layer, which serve as the primary nutrient source for A. muciniphila. Given that carbohydrate chains, linked via O-glycosylation to serines and threonines in the mucin protein backbone, make up to 80% of the molecular weight, it is evident that these O-glycans are important in bacterium's growth and activity[4]. However, the direct relationship between mucin O-glycosylation patterns and the growth of A. muciniphila remains to be established, and how glycoprotein interactions influence this bacterium's abundance.
Diet, as a principal external factor, has a profound influence on the composition and functionality of the gut microbiota, with the microbiome's colonization, growth, and metabolic activities being shaped by dietary constituents. Glycosylated proteins derived from food, such as ovomucin and lactoferrin, have demonstrated significant bioactivities, including anti-inflammatory, antioxidative, anti-tumorigenic properties, and immune system modulation, reflecting the multifaceted roles of dietary glycans in enhancing gut health[5,6]. Recent investigations into the bioactivities of food-derived glycans have shown promising results; for instance, N-glycans purified from human milk not only augmented the proliferation of Bifidobacteria but also highlighted the prebiotic potential of these glycans, alongside indicating their capacity to inhibit pathogenic bacteria and reduce cellular adhesion[7,8]. Given the high degree of N-glycosylation in common dietary proteins from egg and milk, and the substantial variability in protein and glycan chain composition across different foods, it is hypothesized that various foodstuff glycoproteins may differentially affect A. muciniphila's growth and metabolic processes. However, research delineating the specific effects and underlying mechanisms of foodstuff glycoproteins on A. muciniphila remains sparse, underscoring a critical gap in the understanding of dietary influence on this bacterium.
To address these significant questions, the present study employed nonglycosylated recombinant mucin which could bypass the technical problem of deglycosylation of mucin to dissect the influence of mucin's protein and glycan components on the growth of A. muciniphila, aiming to reveal the key factors at play. Furthermore, by examining bovine milk and egg as foodstuff glycoprotein sources, the role of glycosylation in modulating the growth of A. muciniphila was sought. Through comparative analysis of the impacts of various monosaccharides present in O-glycan and N-glycan structures, we began to unravel the differential effects of these glycan types on A. muciniphila (Fig. 1).
Figure 1.
Schematic representation of glycoprotein sources and derivatives influencing the growth of A. muciniphila. Porcine stomach mucin, bovine milk, and egg white/egg yolk serve as sources for glycoproteins and glycans, which are potentially critical for the growth of A. muciniphila within the gut environment. The inset shows a microscopic view of A. muciniphila colonizing the gut mucosa.
-
Escherichia coli Mach1 T1 cells acquired from Life Technologies in Beijing (China) were used for plasmid maintenance, while expression experiments were performed using E. coli BL21 (DE3) strain from Invitrogen, Shanghai. The gene sequence of MUC5AC was synthesized in codon-optimized form for expression in E. coli K12 by Genscript Ltd. (Nanjing, China) as previously described[9]. Additionally, the kits for DNA gel purification and plasmid extraction were sourced from Axygen in Beijing, while peptide substrates were procured from Changzhou Kanglong Biotech Ltd., based in Shanghai, China. Bovine milk and eggs were sourced from Suguo supermarket, Nanjing. All supplementary chemicals utilized throughout this study were of the highest purity and sourced from a series of prominent chemical suppliers within China.
β-elimination to release and purification of mucin O-glycans
-
To a 2 mg sample of porcine stomach mucin (from Shanghai Yuanye Bio-Technology Co., Ltd., Shanghai, China), 500 μL of saturated ammonium hydroxide solution was added. The resulting mixture was incubated at 60 °C for 16 h to facilitate β-elimination. Following incubation, the reaction mixture was subjected to vacuum evaporation to remove the ammonium hydroxide. The sample was subsequently dissolved in ultra-pure water and repeatedly dried to ensure the complete removal of any residual ammonium salt, indicated by the absence of white crystalline solids.
Subsequently, 20 μL of 1% formic acid solution was introduced to the dried sample for acid-catalyzed hydrolysis, conducted in darkness for 40 min. The formic acid was removed by vacuum evaporation. The sample was then reconstituted in 500 μL of ultrapure water, dissolved completely, and centrifuged at 12,000 rpm for 10 min to sediment any insoluble material.
The supernatant, containing the chemically released O-glycans, underwent desalting and purification processes. Initial desalting was done using carbon powder to adsorb contaminants, followed by size exclusion chromatography with a Bio-Gel P-2 column to achieve further purification and separation of purified O-glycans from the sample matrix.
Preparation of nonglycosylated recombinant mucin core protein
-
Given the challenges associated with the chemical deglycosylation of mucin proteins[10], a molecular biology approach was adopted to synthesize a nonglycosylated mimic of human gastric mucin MUC5AC, a substantial glycoprotein with a native molecular weight of approximately 580 kDa. To facilitate expression and purification within an E. coli host system, a truncated SUMO-tagged MUC5AC construct was designed encompassing a specific 45-amino-acid core sequence of the protein. This sequence is representative of the domains found within the full-length protein but is more amenable to bacterial expression systems. The SUMO tag was incorporated to improve protein solubility, increase yield, and ensure proper folding.
Utilizing pSduet-1 as the cloning vector, we engineered the SUMO-MUC5AC expression construct using NcoI and XhoI as cloning sites. The engineered plasmid was transformed into competent E. coli BL21(DE3) ΔlacZ cells, and transformants were selected on agar plates as previously described[11]. A single positive colony was then inoculated into LB medium (containing Kanamycin) and cultured at 37 °C until the optical density measurements at 600 nm (OD600) reached 0.5, indicating mid-log phase growth suitable for protein induction. Recombinant protein expression was induced with 1 mM IPTG, with the culture temperature reduced to 25 °C to facilitate proper protein folding and to reduce the formation of inclusion bodies.
Following a 3-h induction, the cells were harvested and lysed. The lysate was cleared by centrifugation at 12,000 rpm and the supernatant, containing the recombinant protein, was subsequently purified using Ni-NTA affinity chromatography. To remove non-specifically adsorbed proteins, the column-bound material was washed extensively with a buffer that included mild detergents[12]. The bound recombinant protein was then eluted with a buffer containing 500 mM of imidazole, which competes for the metal ions in the column matrix and releases the tagged protein.
The purified protein was then subjected to buffer exchange and concentration using an ultrafiltration device with a 3 kDa molecular weight cutoff. This step was repeated three times to ensure thorough removal of imidazole and other low-molecular-weight components. The final product was lyophilized and stored at −20 °C for subsequent analyses.
Protein identity and purity were verified using matrix-assisted laser desorption/ionization time-of-flight (MALDI-ToF) mass spectrometry, which requires mixing of the protein sample with a sinapinic acid matrix solution. Following co-crystallization on a steel target, the sample was analyzed, and the resulting mass spectra were processed using Bruker Daltonics flex Analysis 3.3 software to determine the mass of the expressed protein component.
Preparation of foodstuff glycoproteins, deglycosylated proteins, and N-glycans
-
To obtain glycoproteins from dairy sources, an initial step involved the centrifugation of 200 mL bovine milk at 12,000 rpm and 4 °C to create a three-layer separation. The middle layer, containing the skim milk with dissolved proteins, was treated with equal volumes of 40% trichloroacetic acid (TCA). For the egg samples, two medium-sized chicken eggs (approx. 50 g/egg) were separated into egg white and egg yolk and were also treated with equal volumes of 40% trichloroacetic acid (TCA). This step was critical for precipitating the proteins and was followed by another round of centrifugation under the same conditions. After discarding the supernatant, the protein pellets were washed and re-centrifuged with water to neutrality (pH 7.0) to ensure that all traces of TCA were removed. The resulting glycoprotein suspensions were subjected to exhaustive dialysis using an 8 kDa molecular weight cut-off membrane for three days to eliminate small molecular weight contaminants. Finally, the glycoprotein samples were lyophilized to produce powdered foodstuff glycoproteins.
For the deglycosylation process, these lyophilized glycoproteins were resolubilized and denatured using 30 mL urea solution (6 M), augmented with 46 mL sodium phosphate buffer (0.5 M, pH 7.0) and 25 mL reductant-containing SDS solution (2%). The mixture was incubated in a boiling water bath to ensure complete denaturation. Once cooled, 38 mL Triton ×100 (10%) and 100 mL enzyme PNGase F were added to specifically cleave N-glycans from the glycoproteins, a process that was carried out at 37 °C over 16 h[13].
The protein-N-glycan mixture was then filtered through ultrafiltration tubes (10 kDa), while centrifugation at low temperature facilitated the separation of the enzyme-deglycosylated proteins from the N-glycans based on molecular size. The ultrafiltration was repeated until the upper layer of the retention solution no longer showed a color when tested with the TLC-based orcinol color reaction, indicating that the N-glycans and deglycosylated proteins were completely separated. Further purification of N-glycans was achieved using carbon powder adsorption and size exclusion chromatography with a Bio-Gel P-2 column to ensure the removal of salts and other impurities.
Before utilization in culturing experiments with A. muciniphila, the glycoproteins, and the resulting deglycosylated proteins were digested enzymatically using pepsin (the reaction volume was 1 L, consisting of 5 g protein, 100 mg pepsin, 2 g NaCl, adjusting pH to 3 with HCl), followed by neutralization and further hydrolysis with trypsin (the reaction volume was 1 L, consisting of 5 g protein, 100 mg trypsin, 6.8 g NaH2PO4, adjusting pH to 7.5 with NaOH). This step was intended to mimic the natural proteolytic digestion conditions within the human gastrointestinal tract, rendering these proteins suitable for evaluating their effect on A. muciniphila growth in an in vitro setting.
Analysis of O-glycan and N-glycan profiles by HILIC-HPLC
-
To verify the composition and structure of O-glycans and N-glycans, the samples were first derivatized with 2-aminobenzamide (2-AB) as previously described[14], a fluorescent marker that enhances detection sensitivity in liquid chromatography. The labeled glycans were then segregated and analyzed by hydrophilic interaction liquid chromatography (Acquity BEH Glycan column 1.7 μm 2.1 mm × 150 mm, Waters) on a Shimadzu Nexera HPLC system equipped with a fluorescence detector. Fluorescent detection parameters were set with an excitation wavelength of 330 nm and an emission wavelength of 420 nm to capture the signature of the 2-AB moiety.
The mobile phases employed for the separation comprised 50 mM ammonium formate, adjusted to pH 4.5 with formic acid, serving as mobile phase A, and chromatographic-grade acetonitrile, which constituted mobile phase B. The stationary phase utilized an Acquity BEH Glycan column (1.7 μm 2.1 mm × 150 mm, Waters) with tightly packed bead resins to ensure resolution of closely related glycan species. The elution gradient began with a high proportion of acetonitrile (95% B) to secure the retention of the glycans on the column, followed by a carefully programmed gradient decrease to 55.9% B over 44.5 min for the elution of the glycans.
The use of this HILIC-HPLC technique not only facilitates the separation of complex glycan mixtures but also enables the comparative assessment of retention times against those of known standard glycans, such as the high-mannose-type N-glycan series obtained from Prozyme. This analytical approach — one that pairs 2-AB labeling with HILIC separation — enables the assessment of potential enzymatic activities by tracking changes in the glycan profile following incubation with glycosidase enzymes, like the recombinant α-mannosidases from Solitalea canadensis or jack bean α-mannosidase[15,16]. By injecting the resultant oligosaccharide mixtures into the HPLC system and observing shifts in peak patterns, researchers can infer enzymatic actions on glycan substrates, elucidating the specificity and efficacy of the enzymes under study.
Optimization of A. muciniphila culture conditions and growth measurements
-
The bacterial strain A. muciniphila strain DSM 22959 was acquired from the German Culture Collection and was employed for growth studies. Preceding the experimental assays, A. muciniphila was subjected to three consecutive culture cycles in brain heart infusion (BHI) medium, composed of 38.5 g/L of the medium (from HaiBo Company, Shandong, China), to ensure culture viability and adaptability. Incubation was conducted at a constant temperature of 37 °C for 48 h.
For growth assays, proteins were administered to the bacterial culture medium at a standardized final concentration of 1 mg/mL. Correspondingly, the quantity of glycans supplemented was equivalent to the amount derived from 1 mg of source glycoprotein. To maintain consistency, an equivalent volume of distilled water served as the negative control for each experiment. Before addition, protein and glycan samples were filter-sterilized using a 0.22-μm nitrocellulose filter to ensure sterility.
The prepared samples were then introduced into 5 mL of fresh BHI broth along with the A. muciniphila culture. The cultures were incubated at 37 °C under anaerobic conditions for 48 h, with bacterial growth progression assessed through its OD600 value. A microphotometer (Onedrop, Nanjing, China) was deployed to obtain these optical density readings at 4-h intervals.
To establish the growth rate (μ) of A. muciniphila under the varying conditions provided by the protein and glycan samples, the following formula was employed:
µ = (lnna − lnn0)ta − t0 Here, n0 and t0 refer to the initial biomass and time, respectively, when the culture enters the logarithmic phase of growth, while na and ta correspond to the biomass and time at the conclusion of the logarithmic phase. By calculating the growth rates, the study aimed to quantify the effects of different proteins and glycans on A. muciniphila growth dynamics to better understand the nutritional preferences and adaptability of the bacterium.
Profiling the glycan consumption by A. muciniphila using HPLC
-
To map the metabolic pathways of A. muciniphila strain DSM 22959 as it ferments specific glycans, we incorporated mucin O-glycans and bovine milk N-glycans into the bacterial growth medium. The objective was to monitor the consumption and utilization of these glycans by the bacteria, as A. muciniphila is known to specialize in mucin degradation within the gut environment.
During the fermentation process, aliquots of the culture were systematically drawn at predetermined intervals to track the temporal changes in glycan concentration. Before analysis, these samples underwent a purification step to remove proteins and other interfering substances, thereby enriching for the glycans of interest. The purification typically involved solid phase extraction using graphitized carbon columns to ensure selective binding and elution of glycans. Before purification, the column was activated with 3 mL of 80% acetonitrile (containing 0.1% TFA) and 3 mL of ultra-pure water. Then, 1 mL of bacterial solution was added, and after the solution flowed through the column by gravity, 3 mL of ultra-pure water was added to elute the salt ions. Finally, 3 mL of 40% acetonitrile (containing 0.1% TFA) was successively added to the column to elute the glycans. Following purification, the glycans were labeled with a fluorescent tag to make them amenable to detection by high-performance liquid chromatography (HPLC). Specific labeling reagents such as 2-AB may be utilized for this purpose, providing a means to visualize the glycans as they separate along the HPLC column. The separation and subsequent analysis were carried out on an HPLC system equipped with a fluorescence detector, where changes in glycan profiles could be observed through the appearance, disappearance, or shift in chromatographic peaks.
By comparing the HPLC profiles of the fermented samples against those of the initial unfermented medium, the metabolic engagement of A. muciniphila with the supplied glycans could be quantitatively and qualitatively assessed. This approach yields important data on the bacterial strain's glycan preferences and the enzymatic efficiency of its glycan-processing repertoire.
Exoglycosidase activity assays
-
The enzymatic activity of exoglycosidases produced by A. muciniphila was evaluated using a set of specific chromogenic substrates, namely pNP-β-GlcNAc, pNP-α-Fuc, pNP-β-Gal, and X-Gal-α-Neu5Ac[17]. These substrates serve as reporters for the activity of various glycoside hydrolases when cleaved, releasing a colorimetric moiety.
Cultures of A. muciniphila were grown at 37 °C for 24 h before the harvest for enzyme assay. Three fractions were prepared from the culture to test for exoglycosidase activities: the sterile supernatant after centrifugation; the washed bacterial pellet for membrane/wall-bound enzymes; and the bacterial lysate for intracellular enzymes.
The sterile supernatant was collected after centrifugation and purified, after which it was directly incubated with the substrate mixture. The bacterial pellet was meticulously washed five times with PBS buffer (pH 7.4) to eliminate any non-specifically adhered components. This pellet was then divided into two aliquots. One aliquot was immediately subjected to enzymatic activity assays to quantify the activity of membrane- or cell wall-associated exoglycosidases, which are accessible from the outside of the bacterial cell.
The second aliquot was resuspended in 500 μL of cell lysis buffer containing 10 μL of 1 mM phenylmethylsulfonyl fluoride (PMSF) to inhibit protease activity. The bacterial cells were subsequently lysed by sonication for 5 min, creating a lysate to assess intracellular exoglycosidase activities.
After the substrate and sample mixtures reacted for 12 h, allowing sufficient time for enzymatic cleavage of the substrates, samples of the supernatant were transferred to a 384-well plate for absorption value detection. The absorbance of the enzymatically released p-nitrophenol was measured at a wavelength of 405 nm using a microplate reader. Comparing the absorbance values across the different substrates and sample fractions provided an overview of the diversity and relative abundance of exoglycosidase activities present in the A. muciniphila cultures.
Effect of monosaccharides on bacterial growth
-
To determine how different monosaccharides influence the growth of A. muciniphila, six representative monosaccharides were selected: N-acetylglucosamine (GlcNAc), N-acetylgalactosamine (GalNAc), galactose, fucose, N-acetylneuraminic acid (Neu5Ac), and mannose. Each monosaccharide was dissolved to achieve a final concentration of 1 mM in solution, and these solutions were then sterilized using a 0.22-μm nitrocellulose filter membrane to ensure that no contaminants would affect the bacterial growth.
A. muciniphila cultures were recovered and then inoculated into 5 mL of BHI medium, constituting 1% of the total culture volume. Simultaneously, the monosaccharide solutions were introduced into the medium, while an equivalent volume of sterile water was added to a control group to serve as a baseline for growth comparison. The various experimental setups, including control and monosaccharide-supplemented groups, were then incubated at a constant 37 °C for 48 h.
Growth dynamics were closely monitored through OD600 values at regular 4-h intervals. These OD600 values reflect the turbidity of the culture medium, which correlates to the cell density and thus provides a measure of the bacterial population growth over time. By comparing the growth curves of A. muciniphila across the different monosaccharide treatments versus the control, the study aimed to elucidate the preference and utilization efficiency of the bacterium for each sugar type tested.
Statistical methods
-
To evaluate the effects of various treatments on the in vitro growth of A. muciniphila and the activities of its exoglycosidases, statistical analyses were employed. The data obtained from these experimental measures were subjected to one-way Analysis of Variance (ANOVA), followed by the Least Significant Difference (LSD) test for multiple comparisons. These analyses were carried out using the SPSS version 21 software package (IBM, Armonk, NY, USA) to determine statistically significant differences among the treatment groups.
For robustness and reliability, all quantitative experiments were conducted in triplicate, providing multiple datasets for each condition tested. Statistical significance was established at a p-value threshold of < 0.05.
-
High-performance liquid chromatography (HPLC) profiling successfully demonstrated the separation of mucin O-glycans released by β-elimination (Fig. 2a). Using a normal-phase HPLC column that elutes compounds based on polarity, the mucin O-glycans predominantly displayed retention times between 5−25 min. The majority of these glycans appeared within the 5−15-min window, suggesting a prevalence of simpler structures, primarily disaccharides to tetrasaccharides. This profile may also hint at side reactions occurring during the β-elimination process, which could potentially fragment and result in partial degradation of the glycan structures.
Figure 2.
HPLC elution profiles of the glycan types investigated. (a) Range of mucin O-glycans by their retention times. (b) Comparison of the retention profiles of N-glycans from bovine milk, egg white, and egg yolk, reinforcing the diversity in N-glycan structures across different food sources.
In contrast, N-glycans, which possess more complex structures compared to O-glycans, exhibited later retention times ranging from 20−40 min (Fig. 2b). The distinct chromatographic profiles of N-glycans derived from bovine milk whey protein, egg white protein, and egg yolk protein highlighted the unique structural attributes of the N-glycans associated with each food source. The discernible disparities among their elution times confirm that these foodstuff glycoproteins harbor unique N-glycan compositions. These findings validate the suitability to utilize these variant N-glycans to investigate their differential impacts on the growth dynamics of A. muciniphila.
Synthesis and characterization of recombinant, nonglycosylated mucin
-
In efforts to dissect the functional role of mucin glycosylation in supporting the growth of A. muciniphila, a recombinant, nonglycosylated version of mucin was used as a nonglycosylated control mucin protein. While the β-elimination method facilitated the release of O-glycans from mucin, this process generally led to the total degradation of the remaining protein constituent, rendering it unusable for subsequent culture experiments. As a workaround, a non-glycosylated version of the mucin protein component - specifically from the MUC5AC isoform - was engineered and expressed in an E. coli system.
The chosen amino acid sequence for the recombinant SUMO-tagged MUC5AC protein encoded a core domain of human MUC5AC (TTPSPVPTTSTTSAPTTSTTSAATTSTISAPTTSAPTTSTTS), characterized by the prevalence of serine and threonine residues. These residues constitute potential O-glycosylation sites in the native protein but remain unmodified in the recombinant form. Following expression and purification, the identity and integrity of the protein were confirmed using Matrix-Assisted Laser Desorption / Ionization Time-of-Flight Mass Spectrometry (MALDI-ToF-MS). As shown in Fig. 3, the observed m/z value for the recombinant SUMO-MUC5AC protein was 18,777.832, which aligned with the theoretical molecular weight, underscoring the accuracy of the expression system and verifying the production of the appropriately sized recombinant protein.
Effects of various glycoproteins, deglycosylated proteins, and glycans on the growth of A. muciniphila
-
Investigations into the influence of mucin-derived products on the growth of A. muciniphila showed that native mucin, its nonglycosylated counterpart SUMO-MUC5AC, and mucin O-glycans contributed to enhanced growth of the bacterium when added to BHI medium (Fig. 4a). Notably, native mucin and its associated O-glycans exerted the most substantial probiotic impact, with mucin-treated cultures exhibiting an extended logarithmic phase, which suggests that mucin constituents provide key nutrients fostering longer periods of sustained bacterial growth. In comparison, the growth promotion by the nonglycosylated mucin SUMO-MUC5AC — rich in threonine, an essential amino acid for A. muciniphila — was also significant relative to the control, particularly evident during the stationary phase of the bacterial lifecycle.
Figure 4.
(a) Comparative effects of mucin O-glycosylation, (b) bovine milk N-glycosylation, (c) egg white N-glycosylation, and (d) egg yolk N-glycosylation on the growth of A. muciniphila, (e) growth rates. A. muciniphila's responses to various glycan and protein substrates are denoted by differing letter groupings, which treatments yielded significant differences in bacterial growth (p < 0.05). (MOG: mucin O-glycan, MUC5AC: nonglycosylated mucin, MGP: bovine milk glycoprotein, MDP: bovine milk deglycosylated protein, MNG: bovine milk N-glycan, WGP: egg white glycoprotein, WDP: egg white deglycosylated protein, WNG: egg white N-glycan, YGP: egg yolk glycoprotein, YDP: egg yolk deglycosylated protein, YNG: egg yolk N-glycan).
Further examinations focused on the impact of foodstuff glycoproteins from milk and eggs, both sources being rich in N-glycosylated proteins. The introduction of bovine milk glycoproteins (MGP) and their deglycosylated forms (MDP) substantially promoted the growth of A. muciniphila (Fig. 4b), attributed to the contribution of amino acids like serine. It was also observed that there was no marked difference in the growth-promoting effect between the intact glycoproteins and their deglycosylated counterparts. This suggests that N-glycosylated motifs from milk proteins may not significantly influence the organism's ability to digest and utilize the proteins. In contrast, bovine milk N-glycans (MNG) appeared to impede the growth of A. muciniphila, as seen from the lesser overall biomass and slower growth rates in comparison to the control group (Fig. 4b). This finding suggests potential regulatory strategies targeting A. muciniphila populations in the gut, considering that excessive levels of this bacterium can lead to mucosal barrier impairment and inflammation[18].
Neither the glycoproteins from egg whites (WGP), their deglycosylated versions (WDP), nor their derived N-glycans (WNG) showed a discernible effect on A. muciniphila growth (Fig. 4c). This could be due to the amino acid profile of egg white proteins not aligning with the preferences of the proteolytic and metabolic systems within A. muciniphila. Despite containing ovomucin, structurally similar to gastrointestinal mucin, its low concentration may explain why supplementation of egg white protein did not significantly promote bacterial growth.
Lastly, no effect on bacterial growth was observed upon addition of glycoproteins, deglycosylated proteins, or N-glycans from egg yolks (Fig. 4d), indicating that not all N-glycans inherently inhibit the growth of A. muciniphila. This result suggests that specific structural properties of bovine milk N-glycans account for their unique inhibitory interaction with A. muciniphila.
Changes in glycan utilization by A. muciniphila during fermentation
-
The capacity of A. muciniphila to metabolize mucin-derived and bovine milk-derived glycans was assessed by monitoring the changes in the glycan composition of the culture supernatant over a 48-hour fermentation period.
A. muciniphila exhibited a comprehensive utilization of mucin O-glycans within the 48-hour fermentation window (Fig. 5a). This observation correlates with the accelerated growth rate of A. muciniphila observed in the presence of mucin O-glycans and suggests that the bacterium possesses a proficient system of glycoside hydrolases capable of extensively hydrolyzing mucin O-glycans. This enzymatic efficiency reflects an evolutionary adaptation of A. muciniphila's glycan-degrading apparatus to the complex carbohydrates present in the gut mucosal environment.
Figure 5.
HPLC profiles that detail A. muciniphila's utilization patterns for (a) mucin O-glycan and (b) bovine milk N-glycan throughout the fermentation process. The depicted chromatograms offer a visual representation of the degree to which A. muciniphila can hydrolyze and assimilate these contrasting glycan types, thus providing insights into the bacterium's substrate specificity and potential nutritional preferences within the intestinal milieu. (MOG: mucin O-glycan, MNG: bovine milk N-glycan).
Conversely, A. muciniphila demonstrated only a marginal utilization of bovine milk N-glycans (Fig. 5b). This limited degradation of bovine milk N-glycan could indicate a relative deficiency in A. muciniphila's enzymatic toolkit for processing the structures present in these particular N-glycans, or an inhibition of growth by undigested glycans.
Impact of glycans on exoglycosidase activity in A. muciniphila
-
Studying the enzymatic activity of A. muciniphila exoglycosidases highlighted noticeable patterns in response to supplementation with mucin O-glycan (MOG) and bovine milk N-glycan (MNG). Overall, no significant differences in the activities of N-acetylglucosaminidase, fucosidase, sialidase, and galactosidase were observed between the MOG group and the control group (p > 0.05) when measured in the BHI-rich-nutrient medium (Fig. 6). This finding suggests that in a nutrient-rich environment, the basal expression levels of A. muciniphila's exoglycosidase genes are sufficient for growth and metabolism.
Figure 6.
Differential activity profiles of A. muciniphila's exoglycosidases when assayed against four synthetic substrates: (a) pNP-β-GlcNAc, (b) pNP-α-Fuc, (c) pNP-β-Gal, and (d) X-Gal-α-Neu5Ac, where variations in enzyme activities are statistically significant (p < 0.05) as indicated by different letters. Comparisons between the various substrate groups reveal nuanced enzyme responses to the presence of glycan molecules, shedding light on A. muciniphila's adaptability in glycan utilization. (MOG: mucin O-glycan, MNG: bovine milk N-glycan).
However, more nuanced insights emerged when probing the secretion levels of certain enzymes. Specifically, N-acetylglucosaminidase and sialidase levels were significantly elevated in the extracellular space of the MOG group compared to the control (p < 0.05) (Fig. 6a & d). This indicates that mucin O-glycan availability influences the distribution of enzyme expression in A. muciniphila, allowing the bacterium to dynamically adapt to the provided nutrients and efficiently upregulate gene expression to facilitate optimal nutrient absorption and metabolic growth. Moreover, the presence of intracellular exoglycosidase activity suggests A. muciniphila's strategy involves importing complex glycan chains into the cell for internal digestion.
In contrast, the MNG group's exoglycosidase activity, with the sole exception of fucosidase, proved significantly lower than that observed in the MOG group and the control group (p < 0.05, Fig. 6). This could be attributed to the intrinsic abundance of fucose modifications within bovine milk N-glycans, which may not negatively impact fucosidase expression. Importantly, the exoglycosidase activities reported here, assessed using p-nitrophenyl (pNP)-linked monosaccharide substrates, may not accurately reflect the enzymes' efficacy against complex glycan chains, which might explain the limited degradation of bovine milk N-glycans seen post-fermentation.
Influence of specific monosaccharides on the growth of A. muciniphila
-
The growth of A. muciniphila was differentially impacted by the presence of various monosaccharides. Certain sugars, such as galactose, GalNAc, GlcNAc, and fucose, were found to act as growth promoters for the bacterium (Fig. 7a−d). With the addition of galactose, a marked increase in bacterial biomass was observable from as early as 12 h into the culture when compared to the control (p < 0.05) (Fig. 7a), underscoring its exceptional probiotic potential for A. muciniphila. GalNAc demonstrated a capacity to swiftly elevate bacterial abundance without leading to overpopulation (Fig. 7b), while both GlcNAc and fucose enhanced the growth rate during the log phase and moderated the decline of bacterial cells during the death phase (Fig. 7c & d).
Figure 7.
Growth curves of A. muciniphila following supplementation with individual monosaccharides—(a) Galactose, (b) GalNAc, (c) GlcNAc, (d) Fucose, (e) Neu5Ac, and (f) mannose—over a time course. (g) Analyses the significant differences in growth observed across the various sugar treatments at each time point. The distinct lettering denotes statistically significant discrepancies between groups (p < 0.05).
Conversely, Neu5Ac and mannose exhibited growth-inhibitory properties toward A. muciniphila, decreasing the growth rate during the logarithmic phase in comparison to the control, and a faster decline phase (Fig. 7e & f). Although Neu5Ac and mannose have negative impacts on the growth of A. muciniphila, the specificity of these effects is noteworthy. The overabundance of A. muciniphila in the gut is implicated in autoimmune responses, and the selective inhibitory action of Neu5Ac and mannose may offer avenues for therapeutic intervention in such conditions[19].
-
A. muciniphila has evolved to not only utilize mucin O-glycan as an adhesion substrate but also as a vital nutrient source[20]. This bacterium predominantly derives its nutrients from mucin[21,22]. Despite this, the individual prebiotic roles played by the protein core and glycan chains within mucin remain unclear. To dissect these roles, recombinant nonglycosylated mucin was synthesized, paving the way for comparing the impacts of native mucin, nonglycosylated mucin, and mucin O-glycan on A. muciniphila's growth dynamics (Fig. 8).
Figure 8.
Workflow illustrating the preparation of glycoproteins, glycans, and deglycosylated proteins from porcine stomach mucin, bovine milk, and egg, followed by the in vitro culture assays of A. muciniphila. The schematic demonstrates the processes, from substrate preparation through the purification steps, to the analysis of microbial growth and metabolic activity in response to different substrates.
This study showed that the inclusion of either mucin or mucin O-glycan significantly enhanced the bacterium's growth compared to other substances (p < 0.05), demonstrating an explicit growth-promoting role attributed to the O-glycan moiety. This suggests that A. muciniphila can decompose and utilize mucin O-glycans[23], and its binding to mucin is O-glycan specific[24]. A. muciniphila also utilized the recombinant MUC5AC mucin protein backbone as a nutrient source. This outcome reveals the preferential utilization of mucin O-glycans by A. muciniphila, likely facilitated by its efficient transport pathways and metabolic networks that optimally incorporate these growth-enhancing factors within the intestinal environment. Unlike Clostridium perfringens, which can only metabolize galactose and fucose when cultured with mucin O-glycan[25], A. muciniphila demonstrated the capability to extensively utilize most components of mucin O-glycan post a 48-h fermentation period. This suggests a uniquely tailored metabolic pathway in A. muciniphila that aligns with mucin O-glycan's structure and composition — a likely result of adaptive evolution to thrive within the mucosal layer of the gut. Furthermore, the effective growth induction by monosaccharides constituting mucin O-glycan, except sialic acid, hints at these monomers serving as preferred substrates that A. muciniphila efficiently catabolizes for carbon and nitrogen sources.
An overgrowth of A. muciniphila in the gut, on the other hand, is linked to health issues, including diarrhea and colorectal cancer[26−28]. With A. muciniphila exhibiting resilience to various antibiotics — some of which may paradoxically encourage its growth[29−31] — the strategies to curb its overabundance are limited. Curiously, the present study shows the possibility that bovine milk N-glycans inhibit A. muciniphila growth, offering an alternative method for modulating its population levels. The observed growth inhibition by bovine milk N-glycan aligns with the documented antibacterial properties of such glycans[13,32,33], potentially tied to their high sialic acid content. The inability of A. muciniphila to use sialic acid as a carbon source, coupled with the acidic nature of this monosaccharide which could depress environmental pH, emerges as a plausible hypothesis for the bacterium's attenuated vitality in its presence. This is in contrast to N-glycans from egg proteins, which lack significant inhibitory effects and are limited to Neu5Ac in composition, whereas bovine milk N-glycan also encapsulates Neu5Gc[34] — a divergence that could account for differential impacts on A. muciniphila growth.
From the point of view of structural complexities, mucin O-glycan's growth-stimulatory effect contrasts with the negligible or adverse impact of foodstuff N-glycans on A. muciniphila. Mucin O-glycans, which contain high amounts of GalNAc, provide a dual source of carbon and nitrogen, enhancing metabolic efficiency. The acetylated amino constituent of GalNAc might also help maintain bacterial cytoplasmic pH homeostasis, reducing the physiological energy expenditure for pH regulation. Similarly, the mannose-rich N-glycans present a challenging matrix for degradation and utilization by A. muciniphila, underscoring the important role of mucin O-glycans as a nutrient source of this bacterium.
-
Both native mucin and free mucin O-glycans can promote the growth of A. muciniphila, with mucin O-glycans identified as the essential component. Remarkably, following a 48-h fermentation period, A. muciniphila was shown to have nearly completely metabolized mucin O-glycan. This comprehensive utilization is accompanied by an increase in extracellular glycosidase activities, signifying the bacterium's ability to adapt and respond to available nutrients in its environment, as well as to regulate gene expression for optimal nutrient absorption and growth.
In contrast to this, N-glycans extracted from egg white and yolk had an insignificant impact on A. muciniphila’s growth. On the other hand, the introduction of bovine milk N-glycan notably inhibited its proliferation, and A. muciniphila displayed minimal utilization of this glycan, suggesting a limiting effect of bovine milk N-glycan on the bacterium's enzyme activities.
In the context of monosaccharides from O-glycans and N-glycans investigated, galactose, GalNAc, GlcNAc, and fucose were favorable for A. muciniphila growth, with galactose pronounced as the most beneficial. In a similar manner, Neu5Ac and mannose were identified as growth inhibitors. The diversity in effects elicited by mucin O-glycan and foodstuff glycoprotein N-glycan on A. muciniphila could be attributed to differences in monosaccharide composition and glycan structure, warranting further insight into their functional roles in the intestinal microbiota.
-
study conception and design: Liu L, Voglmeir J; data collection: Zhang JX, Lv YS; analysis and interpretation of results: Zhang JX, Lv YS. Liu L and Voglmeir J; draft manuscript preparation: Zhang JX. Voglmeir J and Liu L. All authors reviewed the results and approved the final version of the manuscript.
-
All data generated or analyzed during this study are included in this published article.
-
The authors declare that they have no conflict of interest.
- Copyright: © 2024 by the author(s). Published by Maximum Academic Press on behalf of Nanjing Agricultural University. This article is an open access article distributed under Creative Commons Attribution License (CC BY 4.0), visit https://creativecommons.org/licenses/by/4.0/.
-
About this article
Cite this article
Zhang JX, Lyu YS, Voglmeir J, Liu L. 2024. Differential impact of glycoprotein glycosylation on Akkermansia muciniphila growth dynamics. Food Materials Research 4: e022 doi: 10.48130/fmr-0024-0013
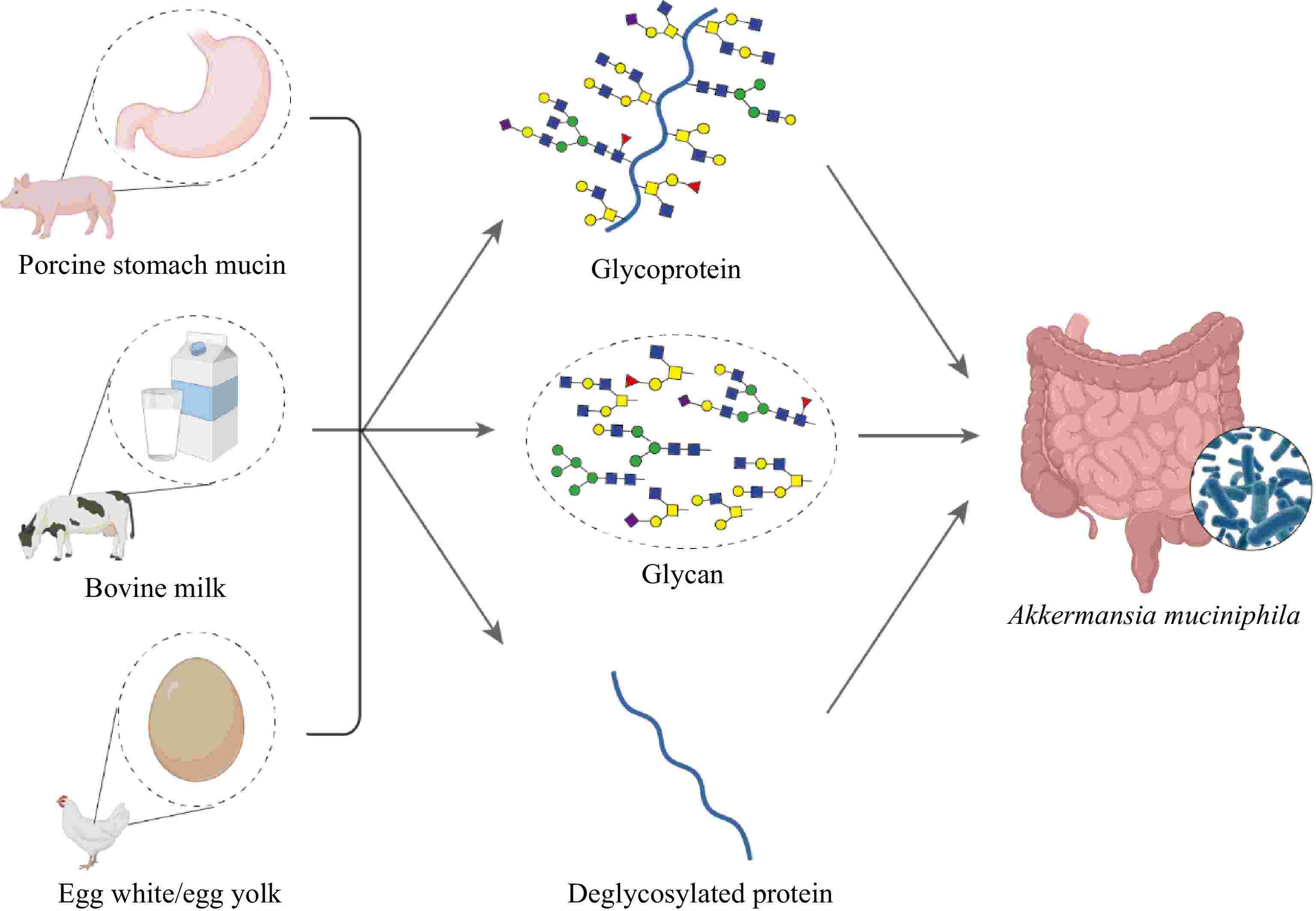